Increased outflow and presence of pharmaceuticals in wastewater from households and hospitals are associated with increased production and consumption of over-the-counter drugs such as painkillers like diclofenac, naproxen, ibuprofen, ketoprofen, or acetaminophen.
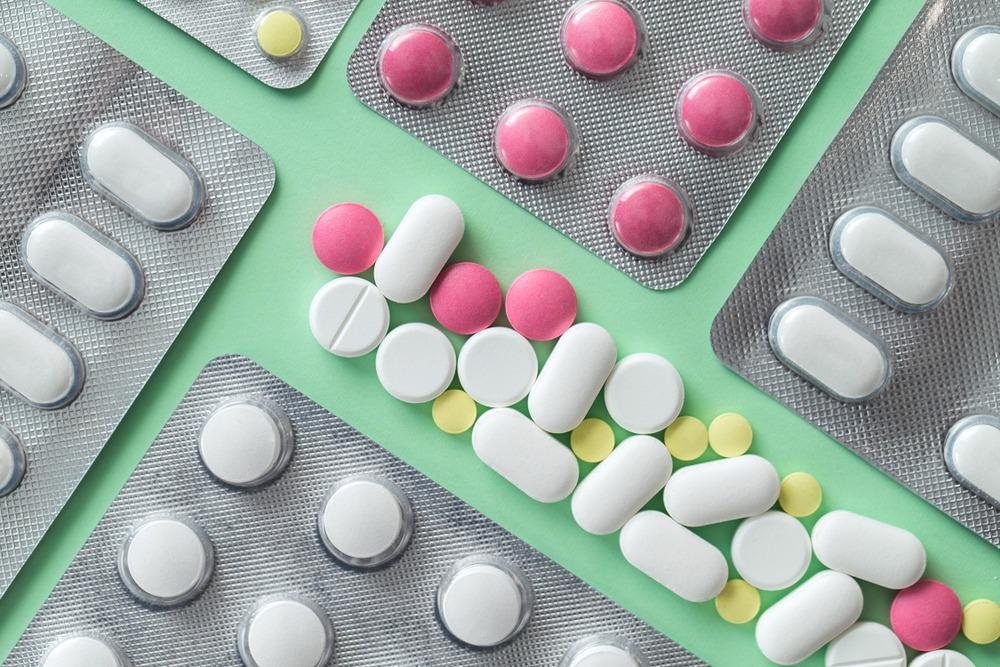
Image Credit: FotoMirta/Shutterstock.com
Certain pharmaceutical residues and sewage end up in wastewater treatment plants (WWTPs), which are ultimately not designed to break down those extremely specialized molecules. However, treated wastewater has been the largest source of pharmaceuticals in the environment. Pharmaceuticals are not removed by conventional WWTPs, hence cleanup rates vary.
Acidic medicines with high water solubility, like acetylsalicylic acid, ibuprofen, ketoprofen, naproxen, and diclofenac (pKa 4.2–4.9), are neither sorbed nor stay in the water. It was discovered that the presence of pharmaceuticals in the water generated severe oxidative stress in Cyprinus Carpio tissues, as well as disturbing microalgal development.
The presence of such compounds in surface waters is harmful to fish and other aquatic species, and it has been linked to an increase in the occurrence of certain diseases, such as cancer (female sex hormones). In research, including 98 pharmaceuticals discovered in various water matrices (treated wastewater, groundwater, and surface water), 11 out of 49 medicines were determined to pose a human health risk when consumed through India’s polluted surface water.
Voltammetric methods are distinguished by their cheap cost, ease of analytic procedure, and ability to collect analytics on the working electrode surface before the suitable electrode process, obviating the need for further concentrating approaches (e.g., the extraction to solid phase).
Screen-printed electrodes (SPEs) were the focus of a slew of studies aiming at determining their practical utility. These electrodes are an appealing analytical instrument because of their inexpensive manufacturing costs, adequate repetition levels, and electrochemical characteristics
Like other electrochemical sensors, the screen-printed electrodes have a small size that allows them to be used in portable/field devices. The use of screen-printed electrodes in in-situ measurements allows for the minimization or even elimination of errors and a reduction in test time and, as a result, expenses associated with the collection, transport, and storage of representative samples.
In recent research published in the MDPI journal sensors, researchers set out to provide a summary of advancements in the area of screen-printed voltammetric sensors used in painkiller environmental water monitoring.
Methodology
Although pharmaceutical concentrations in environmental matrices are typically low—less than 1 μg L−1—authorities are concerned about the long-term effect on animals and people because of their widespread use and abundance in the environment (Table 1).
Table 1. The concentrations and removal rates of painkillers in the environmental matrices. Source: Tyszczuk-Rotko, et al., 2022
Drug |
Excretion and Metabolites |
WWTP Removal Rate
(%) |
Wastewater
Influent
(ng/L) |
Wastewater
Effluent
(ng/L) |
Surface Water
(ng/L) |
diclofenac |
5–10% unchanged, metabolites: glucuronide, sulfate conjugates [49] |
9–60 [50]
57.9 [47] |
up to 302 [50]
191,000 [47] |
1300–3300 [51]
Up to 5450 [50]
10,000 [52]
80,000 [47] |
up to 490 [50]
1200 [48]
1410 [53] |
ibuprofen |
1% unchanged
Metabolites: (+)-2-40-(2-Hydroxy-2-methylpropyl)-phenylpropionic acid (25%) and (+)-2-40-(2- carboxypropyl)-phenylpropionic acid (37%), conjugated ibuprofen (14%) [49] |
78–100 [50]
94.8 [47] |
5533 [50]
344,000 [47] |
711 [50]
18,000 [47] |
400 [50]
126 [53] |
naproxen |
<1 unchanged, metabolites: 6-o-Desmethyl naproxen (o1%), conjugates (66–92%) [49] |
50–98 [50] |
611,000 [50] |
33,900 [50]
10,000 [52] |
297 [53]
390 [48]
400 [50] |
ketoprofen |
Metabolites: Glucuronide conjugates [49] |
15–100 [50] |
5700 [50]
1000–10,000 [54] |
1620 [50] |
120 [48]
329 [50] |
paracetamol |
80% as conjugates, metabolites: Sulphate conjugate (30%), paracetamol cysteinate, mercapturate (5%) [49] |
91–99 [50] |
292,000 [50]
1000–10,000 [54] |
1480 [50]
100,000 [52] |
10,000 [48]
66 [50] |
acetylsalicylic acid |
Metabolites: Salicylic acid (10%), salicyluric acid (75%), salicylic phenolic (10%) and acyl (5%) glucuronides, gentisic acid (o1%) [49] |
0 [50] |
1000–10,000 [54] |
1510 [50] |
<50 [50] |
On the same substrate surface, the whole electrode system (reference, counter, and working electrodes) is imprinted (Figure 1).
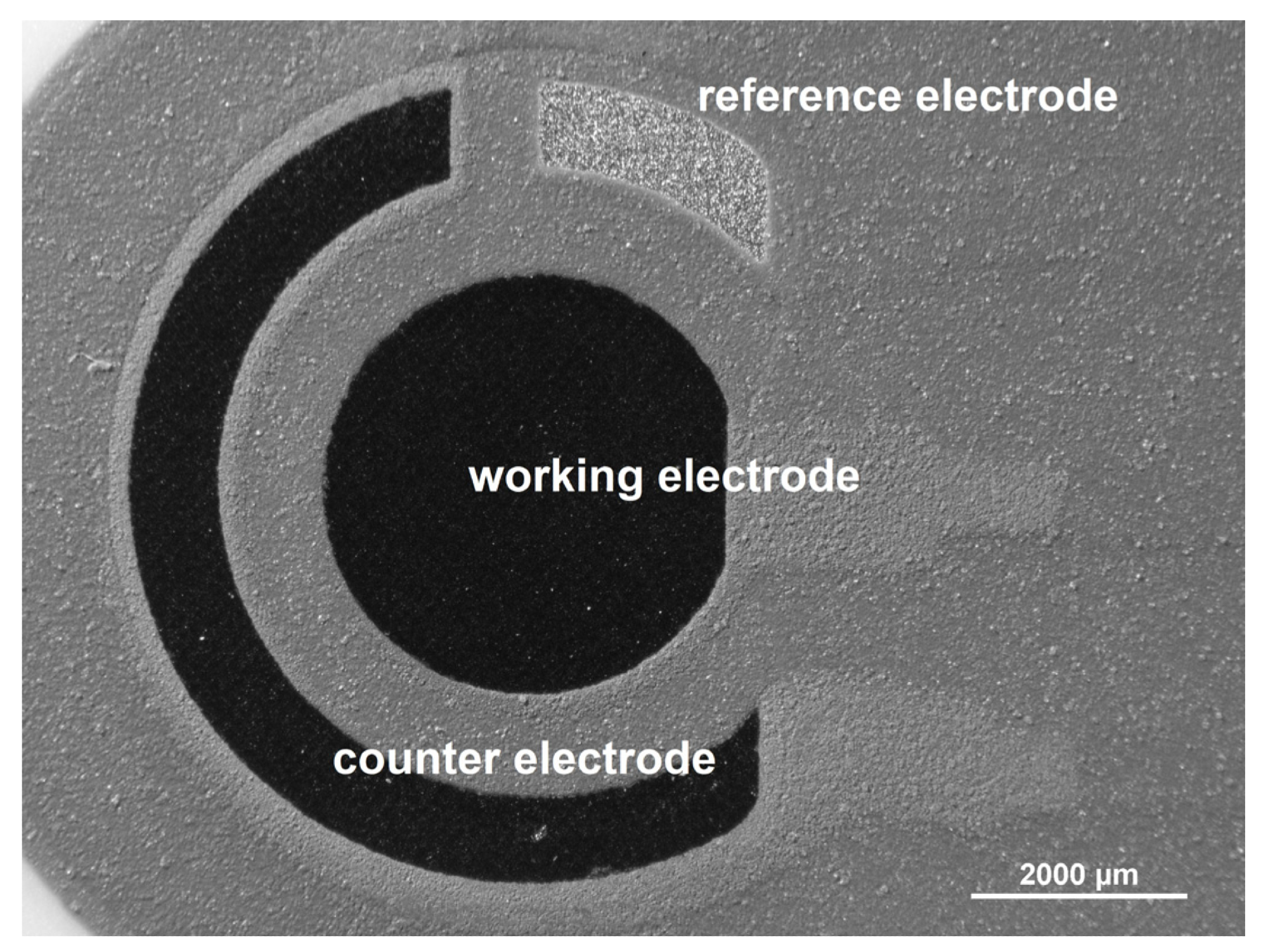
Figure 1. Optical microscopic image of screen-printed carbon electrode (SPCE, Metrohm DropSens, Oviedo, Spain). Image Credit: Tyszczuk-Rotko, et al., 2022
Non-steroidal anti-inflammatory medicines (NSAIDs) are a large pharmacological class since they are commonly used to treat muscular pain and inflammatory rheumatic disorders. Due to increased consumption along with inappropriate disposal and inefficient wastewater treatment, these drugs are commonly found. NSAIDs (Figure 2A–E) include diclofenac (DF), acetylsalicylic acid (AS), ibuprofen (IB), naproxen (NP), and ketoprofen (KP).
Paracetamol (N-acetyl-p-aminophenol, Figure 2F), often known as acetaminophen or Tylenol, is a pain reliever and fever reducer that is widely used across the world. Tramadol is a drug that is used to treat pain (1R, 2R) -2-[(dimethylamino)methyl] -1-(3-methoxyphenyl)cyclohexanol TR, (Figure 2G) is a l-opioid recipient agonist that works centrally on analgesics and is used to treat mild to moderate pain.
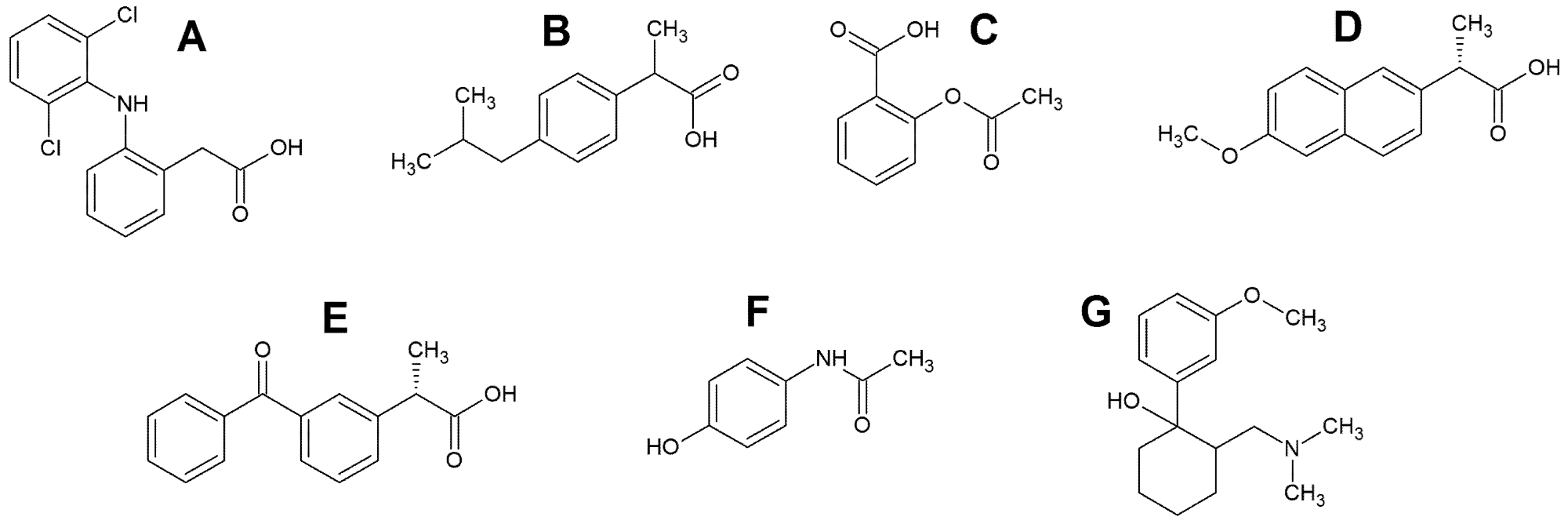
Figure 2. The structural formulas of diclofenac (A), ibuprofen (B), acetylsalicylic acid (C), naproxen (D), ketoprofen (E), paracetamol (F) and tramadol (G). Image Credit: Tyszczuk-Rotko, et al., 2022
Carbon black (CB), carbon nanofibres (CNDs), graphene-related materials, single-, double-, and multiwalled carbon nanotubes (SWCNTs, DWCNTs, and MWCNTs), carbon nanohorns (CNHs), and carbon nano-onions are among the carbon nanomaterials utilized as SPEs modifiers (CNOs).
Few studies in the literature mention the use of screen-printed sensors customized with carbon materials to monitor painkiller residues in water samples. Table 2 presents a comparison of different test techniques.
Table 2. Summary of voltammetric procedures to determine painkillers residues at the screen-printed electrodes modified with carbon materials in environmental water samples. Source: Tyszczuk-Rotko, et al., 2022
Electrode |
Analyte |
Method |
Linear Range
[µM] |
LOD
[µM] |
Application |
Ref. |
SPCE/CNFs |
PA |
DPAdSV |
0.002–0.05
0.1–2.0 |
0.00054 |
river water,
sea water |
[98] |
SPCE/MWCNTs-COOH |
DF |
DPAdSV |
0.0001–0.01 |
0.000028 |
river water |
[102] |
SPCE/MWCNTs-COOH |
PA
DF |
DPAdSV
(PPA) |
0.005–5.0
0.0001–0.02 |
0.0014
0.000030 |
wastewater,
river water |
[83] |
SPCE |
PA |
DPV |
13.20–377.0 |
7.17 |
tap water,
hospital wastewater |
[99] |
SPCNTE |
2.64–33.70 |
0.66 |
SPCNFE |
1.98–33.70 |
0.66 |
SPGPHE |
3.31–23.20 |
0.66 |
SPCE |
IB |
18.40–489.60 |
5.33 |
SPCNTE |
9.21–155.10 |
2.91 |
SPCNFE |
19.40–114.40 |
5.82 |
SPGPHE |
30.50–86.30 |
9.21 |
SPCE |
CF |
24.70–480.0 |
7.21 |
SPCNTE |
20.60–480.0 |
6.18 |
SPCNFE |
61.80–330.0 |
2.06 |
SPGPHE |
15.50–44.80 |
4.63 |
CB/SPCE |
PA
LVF |
SWV |
0.80–30.0
0.90–70.0 |
2.60
0.42 |
river water |
[101] |
PA–paracetamol; DF–diclofenac; IB–ibuprofen; CF–caffeine; LVF–levofloxacin; DPAdSV–differential-pulse adsorptive stripping voltammetry; PPA–pulsed potential accumulation; DPV–differential-pulse voltammetry; SWV–square-wave voltammetry; SPCE–screen-printed carbon electrode; SPCE/MWCNTs-COOH–carboxyl functionalized multiwalled carbon nanotubes modified screen-printed carbon electrode; SPCNTE–screen-printed carbon electrode modified with carbon nanotubes; SPCE/CNFs (SPCNFE)–screen-printed carbon electrode modified with carbon nanofibers; SPGPHE–screen-printed graphene electrode; CB/SPCE–screen-printed carbon electrode modified with carbon black.
Researchers identified a DPAdSV voltammetric technique for the trace measurement of diclofenac (DF) using an available commercially screen-printed carbon sensor altered with carboxyl functionalized multiwalled carbon nanotubes (SPCE/MWCNTs-COOH) (Figure 3).
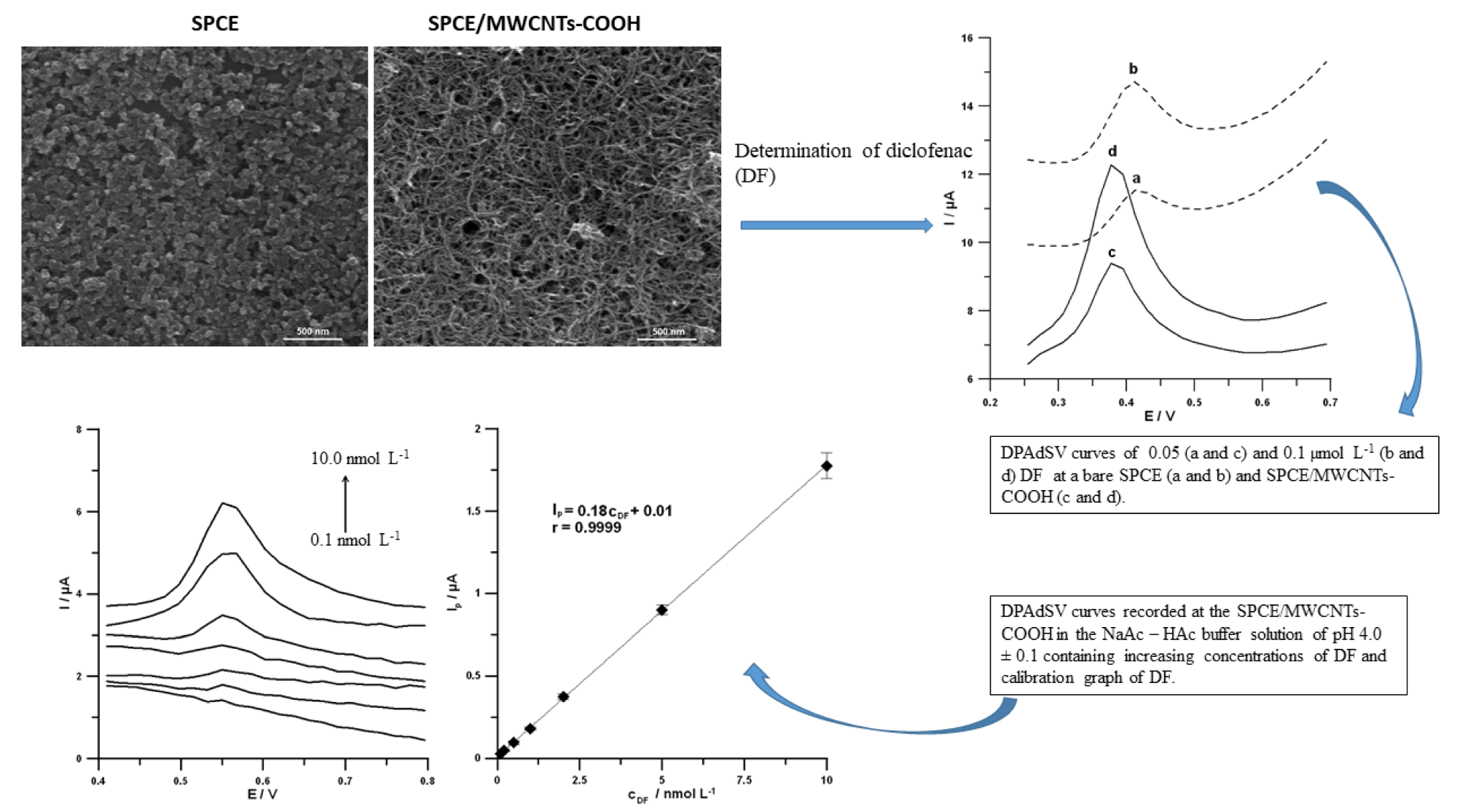
Figure 3. SEM images and DPAdSV curves recorded at the SPCE and SPCE/MWCNTs-COOH. DPAdSV curves recorded at the surface of the SPCE/MWCNTs-COOH in solution containing increasing concentrations of DF: 0.1, 0.2, 0.5, 1.0, 2.0, 5.0 and 10.0 nmol L−1, and calibration graph of DF. Image Credit: Tyszczuk-Rotko, et al., 2022
Figure 4 shows the flowchart of the individual steps of the improved voltammetric technique.
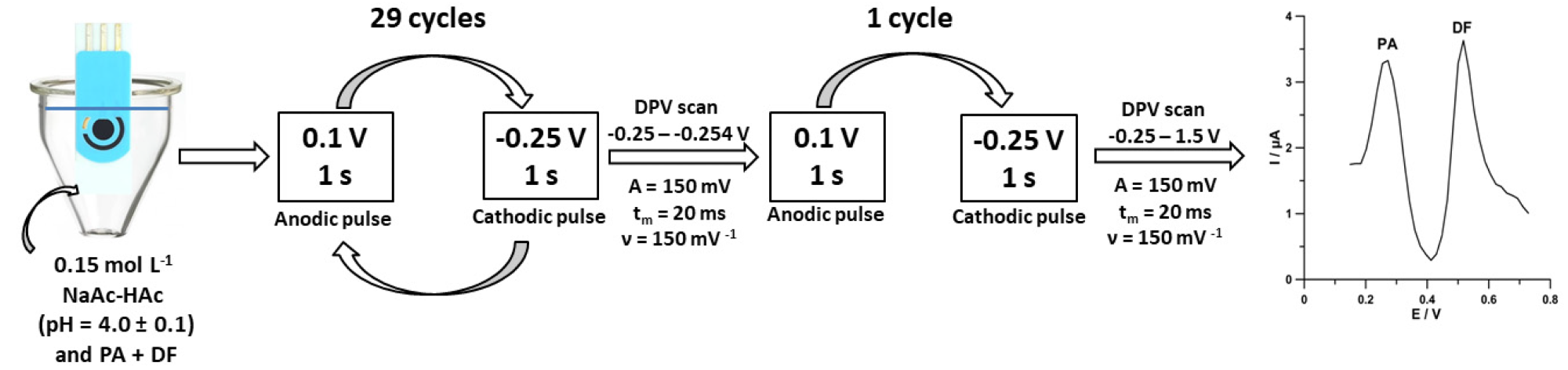
Figure 4. Scheme of voltammetric measurements of PA and DF at the SPCE/MWCNTs-COOH. Image Credit: Tyszczuk-Rotko, et al., 2022
Table 3 lists the voltammetric techniques used to determine painkiller residues on electrochemically prepared screen-printed electrodes.
Table 3. Summary of voltammetric procedures for painkillers residues determination at the electrochemically pretreated screen-printed electrodes or modified with polymer film in environmental water samples. Source: Tyszczuk-Rotko, et al., 2022
Electrode |
Analyte |
Method |
Linear Range
[µM] |
LOD
[µM] |
Application |
Ref. |
aSPCE/SDS |
DF
PA
TR |
DPAdSV |
0.001–0.2
0.05–20.0
0.01–0.2
0.2–2.0 |
0.00021
0.015 |
river water |
[82] |
electrochemically pretreated SPCE |
PA
HQ
E2 |
DPV |
0.5–10.0
0.5–10.0
0.5–10.0 |
0.22
0.19
0.89 |
tap water |
[100] |
electrochemically pretreated SPGE |
IB |
SWV |
0.80–30.0 |
6.30 |
river water,
wastewater |
[104] |
MIP/SPCE |
DF |
DPV |
0.1–10 |
0.07 |
river water,
tap water |
[103] |
PA–paracetamol; DF–diclofenac; HQ–hydroquinone; E2–estradiol; IB–ibuprofen; DPAdSV–differential-pulse adsorptive stripping voltammetry; DPV–differential-pulse voltammetry; SWV–square-wave voltammetry; aSPCE/SDS–activated screen-printed carbon electrode modified with sodium dodecyl sulfate; electrochemically pretreated SPCE–electrochemically pretreated screen-printed carbon electrode; electrochemically pretreated SPGE–electrochemically pretreated screen-printed graphite electrode; MIP/SPCE–screen-printed carbon electrode modified with molecularly imprinted polymer.
Polymers are commonly utilized as electrode and SPE modifiers. Conductive polymers, which combine traditional polymer characteristics with the electrical properties of metals and/or semiconductors, are the most often utilized polymers for this purpose. Polyacetylene, polyaniline, polypyrrole, and polythiophene are the most common materials employed.
Conclusion
Monitoring the water environment for pharmaceutical residues, especially painkiller residues, is a key challenge for modern analytical chemistry. This article shows how screen-printed sensors may be used to detect drug-related environmental water contaminants in a sensitive manner. The devised simple, sensitive, and selective voltammetric processes using screen-printed sensors might be useful instruments for this aim, as shown in several cases in this review paper.
Electroconductivity, catalytic activity, and surface area were all improved when screen-printed electrodes were treated with carbon nanomaterials, polymer film, or electrochemically activated. Furthermore, the screen-printed sensors might be used for in-field analysis as well as laboratory tests.
Screen-printed sensors can be used in environmental water monitoring because of their electrochemical characteristics, simplicity, disposability, rapid reaction time, and compactness.
With new application areas, the screen-printed sensor industry is likely to expand. Future research will concentrate on increasing the analytical characteristics of screen-printed sensors so that they may be adjusted to the relative quantities of analyses in ambient water samples, as well as creating processes and sensors for new analgesic compounds (AS, NP, KP).
Expected interfering species should be given more consideration, as well as known or potential ways for minimizing their impacts and enhancing selectivity. In addition, future research should focus on sensor miniaturization, lowering analysis time, reducing the number of examined samples, and using reagents.
Continue reading: The Applications of Nanoparticles as Synthetic Catalysts.
Journal Reference
Tyszczuk-Rotko, K., Kozak, J. and Czech, B. (2022) Screen-Printed Voltammetric Sensors—Tools for Environmental Water Monitoring of Painkillers. Sensors, 22(7), p.2437. Available Online: https://www.mdpi.com/1424-8220/22/7/2437/htm.
References and Further Reading
- Gaffney, V. J., et al. (2016) Chemical and biochemical characterization and in vivo safety evaluation of pharmaceuticals in drinking water. Environmental and Toxicology Chemistry, 35, pp. 2674–2682. doi.org/10.1002/etc.3451.
- Farré, M.I., et al. (2008) Fate and toxicity of emerging pollutants, their metabolites and transformation products in the aquatic environment. TrAC Trends in Analytical Chemistry, 27, pp. 991–1007. doi.org/10.1016/j.trac.2008.09.010.
- Szymonik, A., et al. (2017) Fate and removal of pharmaceuticals and illegal drugs present in drinking water and wastewater. Ecological Chemistry and Engineering S, 24, 6pp. 5–85. doi.org/10.1515/eces-2017-0006.
- Khan, A. H., et al. (2020) Application of Advanced Oxidation Processes Followed by Different Treatment Technologies for Hospital Wastewater Treatment. Journal of Cleaner Production, 269, p. 122411. doi.org/10.1016/j.jclepro.2020.122411.
- Kosma, C.I., et al. (2010) Occurrence and Removal of PPCPs in Municipal and Hospital Wastewaters in Greece. Journal of Hazardous Materials, 179, pp. 804–817. doi.org/10.1016/j.jhazmat.2010.03.075.
- Angeles, L.F., et al. (2020) Assessing Pharmaceutical Removal and Reduction in Toxicity Provided by Advanced Wastewater Treatment Systems. Environmental Science: Water Research & Technology, 6, pp. 62–77. doi.org/10.1039/C9EW00559E.
- Brillas, E (2022) A Critical Review on Ibuprofen Removal from Synthetic Waters, Natural Waters, and Real Wastewaters by Advanced Oxidation Processes. Chemosphere, 286, p. 131849. doi.org/10.1016/j.chemosphere.2021.131849.
- Larsson, D.G.J., et al. (2007) Effluent from Drug Manufactures Contains Extremely High Levels of Pharmaceuticals. Journal of Hazardous Materials, 148, pp. 751–755. doi.org/10.1016/j.jhazmat.2007.07.008.
- Boroń, M & Pawlas, K (2015) Pharmaceuticals in aquatic environment–literature review. Problemy Higieny i Epidemiologii, 96, p. 357.
- de Morais, J L & Zamora, P P (2005) Use of Advanced Oxidation Processes to Improve the Biodegradability of Mature Landfill Leachates. Journal of Hazardous Materials, 123, pp. 181–186. doi.org/10.1016/j.jhazmat.2005.03.041
- Burns, E.E., et al. (2018) Application of Prioritization Approaches to Optimize Environmental Monitoring and Testing of Pharmaceuticals. Journal of Toxicology and Environmental Health, Part B, 21, pp. 115–141. doi.org/10.1080/10937404.2018.1465873
- Carballa, M., et al. (2007) Fate of Pharmaceutical and Personal Care Products (PPCPs) during Anaerobic Digestion of Sewage Sludge. Water Research, 41, pp. 2139–2150. doi.org/10.1016/j.watres.2007.02.012.
- Evgenidou, E.N., et al. (2015) Occurrence and Removal of Transformation Products of PPCPs and Illicit Drugs in Wastewaters: A Review. Science of The Total Environment, 505, pp. 905–926. doi.org/10.1016/j.scitotenv.2014.10.021.
- Hu, X., et al. (2021) Review on the Role of Plant in Pharmaceuticals and Personal Care Products (PPCPs) Removal in Constructed Wetlands. Science of The Total Environment, 780, p. 146637. doi.org/10.1016/j.scitotenv.2021.146637.
- Fan, X., et al. (2020) Determination of 27 Pharmaceuticals and Personal Care Products (PPCPs) in Water: The Benefit of Isotope Dilution. Frontiers of Environmental Science & Engineering, 14, p. 8. doi.org/10.1007/s11783-019-1187-3.
- OECD (2019) Pharmaceutical Residues in Freshwater: Hazards and Policy Responses. In OECD Studies on Water; OECD: Paris, France.
- Pompei, C.M.E., et al. (2019) Occurrence of PPCPs in a Brazilian Water Reservoir and Their Removal Efficiency by Ecological Filtration. Chemosphere, 226, pp. 210–219. doi.org/10.1016/j.chemosphere.2019.03.122.
- Adeola, A.O., et al. (2021) Adsorption of Antiretroviral Drugs, Efavirenz and Nevirapine from Aqueous Solution by Graphene Wool: Kinetic, Equilibrium, Thermodynamic and Computational Studies. Applied Surface Science Advances, 6, p. 100157. doi.org/10.1016/j.apsadv.2021.100157.
- Babas, H., et al. (2021) Equilibrium and Kinetic Studies for Removal of Antiviral Sofosbuvir from Aqueous Solution by Adsorption on Expanded Perlite: Experimental, Modelling and Optimization. Surfaces and Interfaces, 23, p. 100962. doi.org/10.1016/j.surfin.2021.100962.
- Bhadra, B N & Jhung, S H (2018) Adsorptive Removal of Wide Range of Pharmaceuticals and Personal Care Products from Water Using Bio-MOF-1 Derived Porous Carbon. Microporous and Mesoporous Materials, 270, pp. 102–108. doi.org/10.1016/j.micromeso.2018.05.005.
- Karunanayake, A.G., et al. (2017) Removal of Salicylic Acid, 4-Nitroaniline, Benzoic Acid and Phthalic Acid from Wastewater Using Magnetized Fast Pyrolysis Biochar from Waste Douglas Fir. Chemical Engineering Journal, 319, pp. 75–88. doi.org/10.1016/j.cej.2017.02.116.
- Jafarinejad, S (2017) Cost-Effective Catalytic Materials for AOP Treatment Units. In Applications of Advanced Oxidation Processes (AOPs) in Drinking Water Treatment; Gil, A., Galeano, L.A., Vicente, M.Á., Eds.; Springer International Publishing: Cham, Switzerland, 67, pp. 309–343.
- Michael, I., et al. (2013) Removal of Pharmaceuticals from Environmentally Relevant Matrices by Advanced Oxidation Processes (AOPs). In Comprehensive Analytical Chemistry; Elsevier: Amsterdam, The Netherlands; 62, pp. 345–407. ISBN 978-0-444-62657-8.
- Nie, C., et al. (2016) Fully Solar-Driven Thermo- and Electrochemistry for Advanced Oxidation Processes (STEP-AOPs) of 2-Nitrophenol Wastewater. Chemosphere, 154, pp. 604–612. https://doi.org/10.1016/j.chemosphere.2016.04.020.
- Krishnan, R.Y., et al. (2021) Removal of Emerging Micropollutants Originating from Pharmaceuticals and Personal Care Products (PPCPs) in Water and Wastewater by Advanced Oxidation Processes: A Review. Environmental Technology & Innovation, 23, p. 101757. doi.org/10.1016/j.eti.2021.101757.
- Chevremont, A.-C., et al. (2013) Fate of Carbamazepine and Anthracene in Soils Watered with UV-LED Treated Wastewaters. Water Research, 47, pp. 6574–6584. doi.org/10.1016/j.watres.2013.08.031.
- Pai, C.-W & Wang, G.-S (2022) Treatment of PPCPs and Disinfection By-Product Formation in Drinking Water through Advanced Oxidation Processes: Comparison of UV, UV/Chlorine, and UV/H2O2. Chemosphere, 287, p. 132171. doi.org/10.1016/j.chemosphere.2021.132171.
- Ahmad, N.A., et al. (2015) Removal of Bisphenol A (BPA) in Surface Water by Ozone Oxidation Process. Applied Mechanics and Materials, 735, pp. 210–214. doi.org/10.4028/www.scientific.net/AMM.735.210.
- Beltrán, F.J., et al. (2009) Diclofenac Removal from Water with Ozone and Activated Carbon. Journal of Hazardous Materials, 163, pp. 768–776. doi.org/10.1016/j.jhazmat.2008.07.033.
- Hamdi El Najjar, N., et al. (2014) Kinetics of Paracetamol Oxidation by Ozone and Hydroxyl Radicals, Formation of Transformation Products and Toxicity. Separation and Purification Technology, 136, pp. 137–143. doi.org/10.1016/j.seppur.2014.09.004.
- Lee, C.O., et al. (2012) Ozone and Biofiltration as an Alternative to Reverse Osmosis for Removing PPCPs and Micropollutants from Treated Wastewater. Water Research, 46, pp. 1005–1014. doi.org/10.1016/j.watres.2011.11.069.
- Andreozzi, R., et al. (2003) Paracetamol Oxidation from Aqueous Solutions by Means of Ozonation and H2O2/UV System. Water Research, 37, pp. 993–1004. doi.org/10.1016/S0043-1354(02)00460-8.
- Sharma, J., et al. (2016) Mechanistic Study of Photo-Oxidation of Bisphenol-A (BPA) with Hydrogen Peroxide (H2O2) and Sodium Persulfate (SPS). Journal of Environmental Management, 166, pp. 12–22. doi.org/10.1016/j.jenvman.2015.09.043.
- Abramović, B., et al. (2011) Photocatalytic Degradation of Metoprolol Tartrate in Suspensions of Two TiO2-Based Photocatalysts with Different Surface Area. Identification of Intermediates and Proposal of Degradation Pathways. Journal of Hazardous Materials, 198, pp. 123–132. doi.org/10.1016/j.jhazmat.2011.10.017.
- Bahnemann, D (2004) Photocatalytic Water Treatment: Solar Energy Applications. Journal of Solar energy, 77, pp. 445–459. doi.org/10.1016/j.solener.2004.03.031.
- Guo, W., et al. (2021) Chlorine-Enhanced Photocatalytic Degradation of PPCPs over Bi2MoO6/(BiO)2CO3 Heterostructures. Journal of Environmental Chemical Engineering, 9, p. 106597. doi.org/10.1016/j.jece.2021.106597.
- Kumar, R., et al. (2021) The Removal of Metformin and Other Selected PPCPs from Water by Poly(3,4-Ethylenedioxythiophene) Photocatalyst. Science of The Total Environment, 751, p. 142302. doi.org/10.1016/j.scitotenv.2020.142302.
- Yang, H., et al. (2022) Reducing ROS Generation and Accelerating the Photocatalytic Degradation Rate of PPCPs at Neutral PH by Doping Fe-N-C to g-C3N4. Applied Catalysis B: Environmental, 301, p.120790. https://doi.org/10.1016/j.apcatb.2021.120790.
- Zhang, Q., et al. (2018) A Photocatalytic Degradation Strategy of PPCPs by a Heptazine-Based CN Organic Polymer (OCN) under Visible Light. Environmental Science: Nano, 5, pp. 2325–2336. https://doi.org/10.1039/C8EN00608C.
- Asif, A.H., et al. (2021) Hematite-Based Nanomaterials for Photocatalytic Degradation of Pharmaceuticals and Personal Care Products (PPCPs): A Short Review. Current Opinion in Green and Sustainable Chemistry, 28, 100447. doi.org/10.1016/j.cogsc.2021.100447.
- Qian, H., et al. (2021) Ingenious Control of Adsorbed Oxygen Species to Construct Dual Reaction Centers ZnO@FePc Photo-Fenton Catalyst with High-Speed Electron Transmission Channel for PPCPs Degradation. Applied Catalysis B: Environmental, 291, p. 120064. doi.org/10.1016/j.apcatb.2021.120064.
- Wu, J., et al. (2020) Nanoscale Zero Valent Iron-Activated Persulfate Coupled with Fenton Oxidation Process for Typical Pharmaceuticals and Personal Care Products Degradation. Separation and Purification Technology, 239, p. 116534. https://doi.org/10.1016/j.seppur.2020.116534.
- Savun-Hekimoğlu, B & Ince, N H (2017) Decomposition of PPCPs by Ultrasound-Assisted Advanced Fenton Reaction: A Case Study with Salicylic Acid. Ultrasonics Sonochemistry, 39, pp. 243–249. https://doi.org/10.1016/j.ultsonch.2017.04.013.
- Guo, M., et al. (2021) Enhanced Degradation of Pharmaceuticals and Personal Care Products (PPCPs) by Three-Dimensional Electrocatalysis Coupled Biological Aerated Filter. Journal of Environmental Chemical Engineering, 9, p. 106035. doi.org/10.1016/j.jece.2021.106035.
- Wang, W., et al. (2018) A Microbial Electro-Fenton Cell for Removing Carbamazepine in Wastewater with Electricity Output. Water Research, 139, pp. 58–65. doi.org/10.1016/j.watres.2018.03.066.
- Yang, H., et al. (2018) Rolling-Made Gas Diffusion Electrode with Carbon Nanotube for Electro-Fenton Degradation of Acetylsalicylic Acid. Chemosphere, 206, pp. 439–446. doi.org/10.1016/j.chemosphere.2018.05.027.
- Rashid, S.S & Liu, Y.-Q (2021) Comparison of Life Cycle Toxicity Assessment Methods for Municipal Wastewater Treatment with the Inclusion of Direct Emissions of Metals, PPCPs and EDCs. Science of The Total Environment, 756, p. 143849. doi.org/10.1016/j.scitotenv.2020.143849.
- Boxall, A.B.A., et al. (2004) The Environmental Side Effects of Medication: How Are Human and Veterinary Medicines in Soils and Water Bodies Affecting Human and Environmental Health? EMBO Reports, 5, pp. 1110–1116. doi.org/10.1038/sj.embor.7400307.
- Kasprzyk-Hordern, B., et al. (2008) The Occurrence of Pharmaceuticals, Personal Care Products, Endocrine Disruptors and Illicit Drugs in Surface Water in South Wales, UK. Water Research, 42, pp. 3498–3518. doi.org/10.1016/j.watres.2008.04.026.
- Ziylan, A & Ince, N H (2011) The Occurrence and Fate of Anti-Inflammatory and Analgesic Pharmaceuticals in Sewage and Fresh Water: Treatability by Conventional and Non-Conventional Processes. Journal of Hazardous Materials, 187, pp. 24–36. doi.org/10.1016/j.jhazmat.2011.01.057.
- Stülten, D., et al. (2008) Occurrence of Diclofenac and Selected Metabolites in Sewage Effluents. Science of The Total Environment, 405, pp. 310–316. doi.org/10.1016/j.scitotenv.2008.05.036.
- Wu, D., et al. (2021) Identification of Indicator PPCPs in Landfill Leachates and Livestock Wastewaters Using Multi-Residue Analysis of 70 PPCPs: Analytical Method Development and Application in Yangtze River Delta, China. Science of The Total Environment, 753, p. 141653. doi.org/10.1016/j.scitotenv.2020.141653.
- Al-Baldawi, I.A., et al. (2021) Izzati Application of Phytotechnology in Alleviating Pharmaceuticals and Personal Care Products (PPCPs) in Wastewater: Source, Impacts, Treatment, Mechanisms, Fate, and SWOT Analysis. Journal of Cleaner Production, 319, p. 128584. doi.org/10.1016/j.jclepro.2021.128584.
- Bayati, M., et al. (2021) Assessing the Efficiency of Constructed Wetlands in Removing PPCPs from Treated Wastewater and Mitigating the Ecotoxicological Impacts. International Journal of Hygiene and Environmental Health, 231, p. 113664. doi.org/10.1016/j.ijheh.2020.113664.
- Liu, F., et al. (2014) Effects of Solution Chemistry on Adsorption of Selected Pharmaceuticals and Personal Care Products (PPCPs) by Graphenes and Carbon Nanotubes. Environmental Science and Technology, 48, pp. 13197–13206. doi.org/10.1021/es5034684.
- Stepanova, S., et al. (2013) The Effects of Diclofenac on Early Life Stages of Common Carp (Cyprinus Carpio). Environmental Toxicology and Pharmacology, 35, pp. 454–460. doi.org/10.1016/j.etap.2012.09.011.
- Webb, S., et al. (2003) Indirect human exposure to pharmaceuticals via drinking water. Toxicology Letters, 142, pp. 157–167. doi.org/10.1016/S0378-4274(03)00071-7.
- Maggioni, S., et al. (2013) Screening of endocrine-disrupting phenols, herbicides, steroid estrogens, and estrogenicity in drinking water from the waterworks of 35 Italian cities and from PET-bottled mineral water. Environmental Science and Pollution Research, 20, pp. 1649–1660. doi.org/10.1007/s11356-012-1075-x.
- Brody, J.G., et al. (2006) Breast cancer risk and drinking water contaminated by wastewater: A case control study. Environmental Health, 5, p. 28. doi.org/10.1186/1476-069X-5-28.
- Aschengrau, A., et al. (2011) Affinity for risky behaviors following prenatal and early childhood exposure to tetrachloroethylene (PCE)-contaminated drinking water: A retrospective cohort study. Environmental Health, 10, p. 102. doi.org/10.1186/1476-069X-10-102.
- Zwiener, C (2007) Occurrence and analysis of pharmaceuticals and their transformation products in drinking water treatment. Analytical and Bioanalytical Chemistry, 387, pp. 1159–1162. doi.org/10.1007/s00216-006-0818-2.
- Hena, S., et al. (2021) Removal of Pharmaceutical and Personal Care Products (PPCPs) from Wastewater Using Microalgae: A Review. Journal of Hazardous Materials, 403, p. 124041. doi.org/10.1016/j.jhazmat.2020.124041.
- Sengar, A & Vijayanandan, A (2022) Human health and ecological risk assessment of 98 pharmaceuticals and personal care products (ppcps) detected in indian surface and wastewaters. Science of The Total Environment, 807, p. 150677. doi.org/10.1016/j.scitotenv.2021.150677.
- Kasprzyk-Hordern, B., et al. (2007) Occurrence of acidic pharmaceuticals in the Warta River in Poland. Chemia Analityczna, 52, pp. 289–303.
- Caban, M., et al. (2015) Determination of pharmaceutical residues in drinking water in Poland using a new SPE-GC-MS(SIM) method based on Speedisk extraction disks and DIMETRIS derivatization. Science of The Total Environment, 538, pp. 402–411. doi.org/10.1016/j.scitotenv.2015.08.076.
- Mirasole, C., et al. (2016) Liquid chromatography–tandem mass spectrometry and passive sampling: Powerful tools for the determination of emerging pollutants in water for human consumption. Journal of Mass Spectrometry, 51, pp. 814–820. doi.org/10.1002/jms.3813.
- Ma, R., et al. (2016) Characterization of pharmaceutically active compounds in Dongting Lake, China: Occurrence, chiral profiling and environmental risk. Science of The Total Environment, 268, pp. 557–558. doi.org/10.1016/j.scitotenv.2016.03.053.
- Paíga, P., et al. (2017) Development of a multi-residue method for the determination of human and veterinary pharmaceuticals and some of their metabolites in aqueous environmental matrices by SPE-UHPLC-MS/MS. Journal of Pharmaceutical and Biomedical Analysis, 135, pp. 75–86. doi.org/10.1016/j.jpba.2016.12.013.
- Wang, J (1985) Stripping Analysis, Principles, Instrumentation and Applications; VCH Publishers: Hoboken, NJ, USA.
- Couto, R.A.S., et al. (2016) Recent developments, characteristics and potential applications of screen-printed electrodes in pharmaceutical and biological analysis. Talanta, 146, pp. 801–814. doi.org/10.1016/j.talanta.2015.06.011.
- Barton, J., et al. (2016) Screen-printed electrodes for environmental monitoring of heavy metal ions: A review. Microchimica Acta, 183, pp. 503–517. doi.org/10.1007/s00604-015-1651-0.
- Niu, X., et al. (2013) Electrochemical Stripping Analysis of Trace Heavy Metals Using Screen-Printed Electrodes. Analytical Letters, 46, pp. 2479–2502. doi.org/10.1080/00032719.2013.805416
- Trojanowicz, M (2016) Impact of nanotechnology on design of advanced screen-printed electrodes for different analytical applications. TrAC Trends in Analytical Chemistry, 84, pp. 22–47. doi.org/10.1016/j.trac.2016.03.027.
- Arduini, F., et al. (2016) Electrochemical biosensors based on nanomodified screen-printed electrodes: Recent applications in clinical analysis. TrAC Trends in Analytical Chemistry, 79, pp. 114–126. doi.org/10.1016/j.trac.2016.01.032.
- Hughes, G., et al. (2016) Recent advances in the fabrication and application of screen-printed electrochemical (bio) sensors based on carbon materials for biomedical, agri-food and environmental analyses. Biosensors, 6, p. 50. doi.org/10.3390/bios6040050.
- Cinti, S & Arduini, F (2017) Graphene-based screen-printed electrochemical (bio)sensors and their applications: Efforts and criticisms. Biosensors and Bioelectronics, 89, p. 107122. doi.org/10.1016/j.bios.2016.07.005.
- Tyszczuk-Rotko, K., et al. (2017) Green Electrochemical Sensor for Caffeine Determination in Environmental Water Samples: The Bismuth Film Screen-Printed Carbon Electrode. Journal of The Electrochemical Society, 164, p. B342. doi.org/10.1149/2.0571707jes.
- Hayat, A & Marty, J L (2014) Disposable Screen Printed Electrochemical Sensors: Tools for Environmental Monitoring. Sensors, 14, pp. 10432–10453. doi.org/10.3390/s140610432.
- Namieśnik, J (2001) Modern Trends in Monitoring and Analysis of Environmental Pollutants. Polish Journal of Environmental Studies, 10, pp. 127–140.
- Feier, B., et al. (2018) Electrochemical detection and removal of pharmaceuticals in waste waters. Current Opinion in Electrochemistry, 11, pp. 1–11. doi.org/10.1016/j.coelec.2018.06.012.
- Torrinha, Á., et al. (2021) Carbon paper as a promising sensing material: Characterization and electroanalysis of ketoprofen in wastewater and fish. Talanta, 226, p. 122111. doi.org/10.1016/j.talanta.2021.122111
- Kozak, J., et al. (2021) Electrochemically Activated Screen-Printed Carbon Sensor Modified with Anionic Surfactant (aSPCE/SDS) for Simultaneous Determination of Paracetamol, Diclofenac and Tramadol. Materials, 14, p. 3581. doi.org/10.3390/ma14133581.
- Sasal, A., et al. (2020) Simultaneous analysis of paracetamol and diclofenac using MWCNTs-COOH modified screen-printed carbon electrode and pulsed potential accumulation. Materials, 13, 3091. doi.org/10.3390/ma13143091.
- Jahani, P.M., et al. (2020) Simultaneous voltammetric detection of morphine and diclofenac using graphene nanoribbon modified screen-printed electrode. International Journal of Electrochemical Science, 15, pp. 9037–9048. doi.org/10.20964/2020.09.14.
- Zhang, C., et al. (2020) electrochemical sensor based on plasma-treated zinc oxide nanoflowers for the simultaneous detection of dopamine and diclofenac sodium. Microchemical Journal, 158, p. 105237. doi.org/10.1016/j.microc.2020.105237.
- Kimuama, K., et al. (2020) Single step preparation of platinum nanoflowers/reduced graphene oxide electrode as a novel platform for diclofenac sensor. Microchemical Journal, 155, p. 104744. doi.org/10.1016/j.microc.2020.104744.
- Tyszczuk-Rotko, K., et al. (2021) Electrochemically activated screen-printed carbon electrode for determination of ibuprofen. Applied Sciences, 11, p. 9908. doi.org/10.3390/app11219908.
- Bushra, R & Aslam, N (2010) An overview of clinical pharmacology of ibuprofen. Oman Medical Journal, 25, pp. 155–161. doi.org/10.5001/omj.2010.49.
- Diouf, A., et al. (2010) An electrochemical sensor based on chitosan capped with gold nanoparticles combined with a voltammetric electronic tongue for quantitative aspirin detection in human physiological fluids and tablets. Materials Science Engineering, 110, p.110665. doi.org/10.1016/j.msec.2020.110665.
- Kruanetr, S., et al. (2015) The electrochemical detection of aspirin utilising screen printed Graphene electrodes as sensors platforms. Surface Engineering and Applied Electrochemistry, 51, pp. 283–289. doi.org/10.3103/S1068375515030114.
- Stefano, J.S., et al. (2014) Flow-injection analysis with multiple-pulse amperometry for simultaneous determination of paracetamol and naproxen using a homemade flow cell for screen-printed electrodes. Journal of the Brazilian Chemical Society, 25, pp. 484–491. doi.org/10.5935/0103-5053.20140006.
- Kondori, T., et al. (2021) Synthesis and characterization of bipyridine cobalt(II) complex modified graphite screen printed electrode: An electrochemical sensor for simultaneous detection of acetaminophen and naproxen. RSC Advances, 11, pp. 3049–3057. doi.org/10.1039/D0RA08126D.
- Kuczyńska, J & Nieradko-Iwanicka, B (2021) The effect of ketoprofen lysine salt on mucosa of rat stomach after ethyl alcohol intoxication. Biomedicine & Pharmacotherapy, 141, p. 111938. doi.org/10.1016/j.biopha.2021.111938.
- Molina-Garcia, L., et al. (2013) Determination of ketoprofen based on its quenching effect in the fluorescence of quantum dots. Journal of Food and Drug Analysis, 21, pp. 426–431. doi.org/10.1016/j.jfda.2013.09.007.
- Cao, F., et al. (2018) Electrochemical sensor for detecting pain reliever/fever reducer drug acetaminophen based on electrospun CeBiOx nanofibers modified screen-printed electrode. Sensors and Actuators B: Chemical, 256, 143–150. doi.org/10.1016/j.snb.2017.09.204.
- Jahani, P.M., et al. (2020) Simultaneous voltammetric detection of acetaminophen and tramadol using molybdenum tungsten disulfide-modified graphite screen-printed electrode. International Journal of Electrochemical Science, 15, pp. 9024–9036. doi.org/10.20964/2020.09.12.
- Sima, V., et al. (2010) Screen-printed electrodes modified with HRP-zirconium alkoxide film for the development of a biosensor for acetaminophen detection. Central European Journal of Chemistry, 8, pp. 1034–1040.
- Sasal, A., et al. (2020) Direct determination of paracetamol in environmental samples using screen-printed carbon/carbon nanofibers sensor—Experimental and theoretical studies. Electroanalysis, 32, pp. 1618–1628. doi.org/10.1002/elan.202000039.
- Serrano, N., et al. (2019) Commercial screen-printed electrodes based on carbon nanomaterials for a fast and cost-effective voltammetric determination of paracetamol, ibuprofen and caffeine in water samples. Sensors, 19, p. 4039. doi.org/10.3390/s19184039.
- Raymundo-Pereira, P.A., et al. (2019) Simultaneous, ultrasensitive detection of hydroquinone, paracetamol and estradiol for quality control of tap water with a simple electrochemical method. Journal of Electroanalytical Chemistry, 848, p. 113319. doi.org/10.1016/j.jelechem.2019.113319.
- Deroco, P.B., et al. (2019) Effect of different carbon blacks on the simultaneous electroanalysis of drugs as water contaminants based on screen-printed sensors. Electroanalysis, 31, pp. 2145–2154. doi.org/10.1002/elan.201900042.
- Sasal, A., et al. (2020) First electrochemical sensor (screen-printed carbon electrode modified with carboxyl functionalized multiwalled carbon nanotubes) for ultratrace determination of diclofenac. Materials, 13, p. 781. doi.org/10.3390/ma13030781.
- Seguro, I., et al. (2021) Low cost, easy to prepare and disposable electrochemical molecularly imprinted sensor for diclofenac detection. Sensors, 21, p. 1975. doi.org/10.3390/s21061975.
- Amin, S., et al. (2014) Disposable screen printed graphite electrode for the direct determination of ibuprofen in surface water. Environmental Nanotechnology, Monitoring and Management, 1–2, pp. 8–13.
- Saciloto, T.R., et al. (2013) Simultaneous voltammetric determination of acetaminophen and caffeine at a graphite and polyurethane screen-printed composite electrode. Journal of the Brazilian Chemical Society, 24, pp. 1461–1468. doi.org/10.5935/0103-5053.20130186.
- Gilmartin, M.A.T., et al. (1994) Rapid detection of paracetamol using a disposable, surface-modified screen-printed carbon electrode. Analyst, 119, pp. 2431–2437. doi.org/10.1039/AN9941902431.
- Ma, L.-L., et al. (2021) Fabrication, characterization and performance evaluation of screen-printed carbon electrodes: Determination of acetaminophen in Tylenol. Chinese. Chinese Journal of Analytical Chemistry, 49, pp. 21187–21196. doi.org/10.1016/S1872-2040(21)60116-0.
- Khairy, M & Banks, C.E (2020) A screen-printed electrochemical sensing platform surface modified with nanostructured ytterbium oxide nanoplates facilitating the electroanalytical sensing of the analgesic drugs acetaminophen and tramadol. Microchimica Acta, 187, p. 126. doi.org/10.1007/s00604-020-4118-x.
- Zhang, Y., et al. (2019) Simultaneous voltammetric determination of acetaminophen and isoniazid using MXene modified screen-printed electrode. Biosensors and Bioelectronics, 130, pp. 315–321. doi.org/10.1016/j.bios.2019.01.043.
- Wei, Z., et al. (2019) Simultaneous determination of acetaminophen and tyrosine using screen-printed electrochemical sensor based on MWCNTs-doped poly(glycine)/poly(acrylic acid) conducting polymers. International Journal of Electrochemical Science, 14, pp. 6748–6758. doi.org/10.20964/2019.07.26.
- De Carvalhoa, R.C., et al. (2020) Diclofenac determination using CeO2 nanoparticle modified screen-printed electrodes—A study of background correction. Microchemical Journal, 158, pp. 105258. doi.org/10.1016/j.microc.2020.105258.
- Baezzat, M.R., et al. (2022) Construction of a new electrochemical sensor based on MoS2 nanosheets modifiedgraphite screen printed electrode for simultaneous determination of diclofenac and morphine. Analytical and Bioanalytical Chemistry Research, 9, pp. 153–162.
- Apetrei, I.M., et al. (2017) Determination of ibuprofen based on screen-printed electrodes modified with carbon nanofibers. Farmacia, 65, pp. 790–795.
- Zhao, C & Lin, J (2017) Electrochemically reduced graphene oxide modified screen-printed electrodes for sensitive determination of acetylsalicylic acid. International Journal of Electrochemical Science, 12, pp. 10177–10186. doi.org/10.20964/2017.11.03.
- Baj-Rossi, C., et al. (2014) Continuous monitoring of Naproxen by a cytochrome P450-based electrochemical sensor. Biosensors and Bioelectronics, 53, pp. 283–287. doi.org/10.1016/j.bios.2013.09.058.
- Yaghoubian, H., et al. (2014) Fe2MoO4 magnetic nanocomposite modified screenprinted graphite electrode as a voltammetric sensor for simultaneous determination of nalbuphine and diclofenac. Journal of Materials Science: Materials in Electronics, 32, pp. 17311–17323. doi.org/10.1007/s10854-021-06244-3.
- Cumba, L.R., et al. (2020) Electrochemical properties of screen-printed carbon nano-onion electrodes. Molecules, 25, p. 3884. doi.org/10.3390/molecules25173884.
- Kozak, J., et al. (2022) Application of a screen-printed sensor modified with carbon nanofibers for the voltammetric analysis of an anticancer disubstited fused triazinone. International Journal of Molecular Sciences, 23, p. 2429. doi.org/10.3390/ijms23052429.
- Ibáñez-Redín, G., et al. (2019) Screen-printed interdigitated electrodes modified with nanostructured carbon nano-onion films for detecting the cancer biomarker CA19-9. Materials Science and Engineering: C, 99, pp. 1502–1508. doi.org/10.1016/j.msec.2019.02.065.
- Kaewket, K., et al. (2021) Anti-fouling effects of carbon nanofiber in electrochemical sensing of phenolic compounds. Journal of The Electrochemical Society, 168, p. 067501. doi.org/10.1149/1945-7111/ac0358.
- Della Pelle, F., et al. (2018) Nano carbon black-based screen-printed sensor for carbofuran, isoprocarb, carbaryl and fenobucarb detection: Application to grain samples. Talanta, 186, pp. 389–396. doi.org/10.1016/j.talanta.2018.04.082.
- Bounegru, A V & Apetrei, C (2021) Voltamperometric sensors and biosensors based on carbon nanomaterials used for detecting of caffeic acid-a review. International Journal of Molecular Sciences, 21, p. 9275. doi.org/10.3390/ijms21239275.
- Sipa, K., et al. (2019) The application of carbon nanomaterials as electrode surface modifiers for the voltammetric sensing of nitroxinil—A comparative studies. Journal of Electroanalytical Chemistry, 848, p. 113294. doi.org/10.1016/j.jelechem.2019.113294.
- Goyal, R.N., et al. (2010). Electrochemical investigations of diclofenac at edge plane pyrolytic graphite electrode and its determination in human urine. Sensors and Actuators B: Chemical, 145, pp. 743–748. doi.org/10.1016/j.snb.2010.01.038.
- Medsen, K.G., et al. (2008) Bioactivation of diclofenac in vitro and In Vivo: Correlation to electrochemical studies. Chemical Research in Toxicology, 21, 1107–1119. doi.org/10.1021/tx700419d
- Korolczuk, M (2000) Application of pulsed potential accumulation for minimization of interferences from surfactants in voltammetric determination of traces of Cr(VI). Electroanalysis, 12, 837–840. doi.org/10.1002/1521-4109(200007)12:11<837::AID-ELAN837>3.0.CO;2-D.
- González-Sánchez, M.I., et al. (2018) Highly activated screen-printed carbon electrodes by electrochemical treatment with hydrogen peroxide. Electrochemistry Communications, 91, pp. 36–40. doi.org/10.1016/j.elecom.2018.05.002.
- Yuan, X., et al. (2021) Simple pre-treatment by low-oxygen plasma activates screen-printed carbon electrode: Potential for mass production. Applied Surface Science, 544, p. 148760. doi.org/10.1016/j.apsusc.2020.148760.
- Wei, H., et al. (2007) Enhanced electrochemical performance at screen-printed carbon electrodes by a new pretreating procedure. Analytica Chimica Acta, 588, pp. 297–303. doi.org/10.1016/j.aca.2007.02.006.
- Lee, J., et al. (2016) Mechanical polishing as an improved surface treatment for platinum screen-printed electrodes. Sensing and Bio-Sensing Research, 9, pp. 38–44. doi.org/10.1016/j.sbsr.2016.05.006.
- Cumba, L.R., et al. (2016) Can the mechanical activation (polishing) of screen-printed electrodes enhance their electroanalytical response? Analyst, 141, pp. 2791–2799. doi.org/10.1039/C6AN00167J.
- Montiel, N.F., et al. (2021) The opportunity of 6-monoacetymorphine to selectively detect heroin at prenodized screen printed electrodes. Talanta, 226, 122005. doi.org/10.1016/j.talanta.2020.122005.
- De Oliveira Silva, R., et al. (2019) Electrochemically activated multiwalled carbon nanotubes modified screen-printed electrode for voltammetric determination of sulfentrazone. Journal of Electroanalytical Chemistry, 835, pp. 220–226. doi.org/10.1016/j.jelechem.2019.01.018.
- Kozak, J., et al. (2021) First screen-printed sensor (electrochemically activated screen-printed boron-doped diamond electrode) for quantitative determination of rifampicin by adsorptive stripping voltammetry. Materials, 14, p. 4231. doi.org/10.3390/ma14154231.
- González-Sánchez, M. I., et al. (2019) Electrochemical performance of activated screen-printed carbon electrodes for hydrogen peroxide and phenol derivatives sensing. Journal of Electroanalytical Chemistry, 839, pp. 75–82. doi.org/10.1016/j.jelechem.2019.03.026.
- Cinto, S (2017) Polymeric materials for printed-based electroanalytical (bio)applications. Chemosensors, 5, p. 31. doi.org/10.3390/chemosensors5040031.
- Raj, M., et al. (2017) Graphene/conducting polimer nano-composite loaded screen printed carbon sensor for simultaneous determination of dopaminę and 5-hydroxytryptamine. Sensors and Actuators B: Chemical, 239, pp. 993–1002. doi.org/10.1016/j.snb.2016.08.083.
- Valasii, L., et al. (2015) Disposable nafion-modified screen-printed graphite electrodes for the rapid voltammetric assay of caffeine. Insights in Analytical Electrochemistry, 1, pp. 2470–9867.
- Kumar, D & Prasad, B B (2012) Multiwalled carbon nanotubes embedded molecularly imprinted polimer-modified screen printed carbon electrode for the quantitative analysis of C-reactive protein. Sensors and Actuators B: Chemical, 171–172, pp. 1141–1150. doi.org/10.1016/j.snb.2012.06.053.
- Stoica, B.E., et al. (2021) Uncovering the behavior of screen-printed carbon electrodes modified with polymers molecularly imprinted with lipopolysaccharide. Electrochemistry Communications, 124, p. 106965. doi.org/10.1016/j.elecom.2021.106965.
- Ekomo, V.M., et al. (2018) Detection of Bisphenol A in aqueous medium by screen printed carbon electrodes incorporating electrochemical molecularly imprinted polymers. Biosensors and Bioelectronics, 112, pp. 156–161. doi.org/10.1016/j.bios.2018.04.022.
- Ayankojo, A.G., et al. (2020) Sulfamethizole-imprinted polymer on screen-printed electrodes: Towards the design of a portable environmental sensor. Sensors and Actuators B: Chemical, 320, p. 128600. doi.org/10.1016/j.snb.2020.128600.
- Rebelo, P., et al. (2020) Azithromycin electrochemical detection using a molecularly imprinted polymer prepared on a disposable screen-printed electrode. Analytical Methods, 12, pp. 1486–1494. doi.org/10.1039/C9AY02566A.
- Motaharian, A., et al. (2019) Determination of psychotropic drug chlorpromazine using screen printed carbon electrodes modified with novel MIP-MWCNTs nano-composite prepared by suspension polymerization method. Sensors and Actuators B: Chemical, 288, pp. 356–362. doi.org/10.1016/j.snb.2019.03.007.
- Antiochia, R & Gorton, L (2014) A new osmium-polymer modified screen-printed graphene electrodefor fructose detection. Sensors and Actuators B: Chemical, 195, pp. 287–293. doi.org/10.1016/j.snb.2014.01.050.
- Chakkarapani, L D & Brandl, M (2020) Carbon screen-printed electrode coated with poly(toluidine blue) as an electrochemical sensor for the detection of tyramine. Engineering Proceedings, 2, pp. 51–56.
- Faradilla, P., et al. (2022) Electrochemical sensor based on screen printed carbon electrode–zinc oxide nano particles/molecularly imprinted-polymer (SPCE–ZnONPs/MIP) for detection of sodium dodecyl sulfate (SDS). RSC Advances, 12, pp. 743–752. doi.org/10.1039/D1RA06862H.