For electrochemical trace analysis, improved electrochemical sensors with enhanced sensitivity and low detection limits are in great demand. Electrochemical sensing performance is primarily determined by the characteristics of electrode materials and the general electrode shape.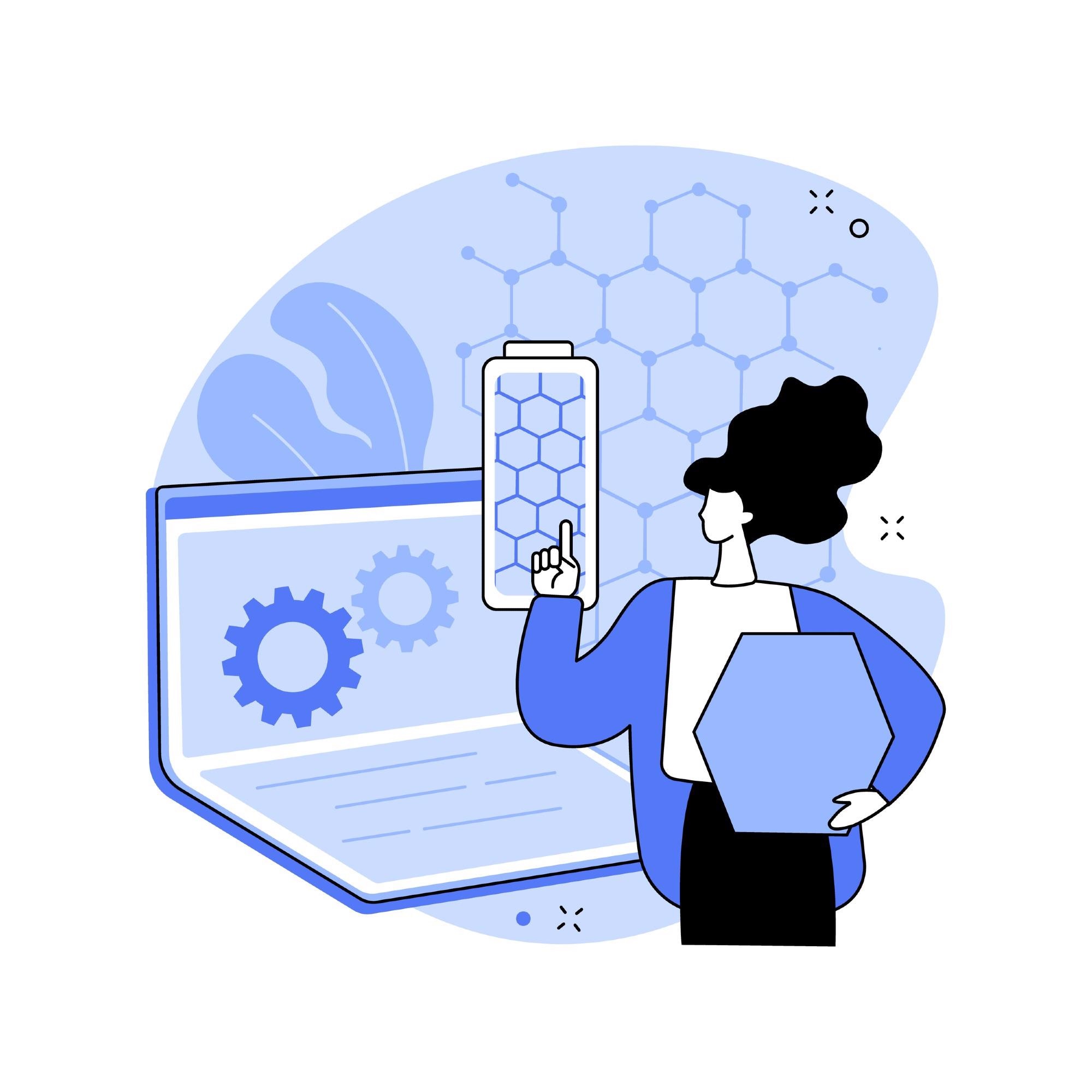
Image Credit: Visual Generation/Shutterstock.com
High electrochemical sensitivity has been achieved by using graphene, a 2-dimensional (2D) nanocarbon structure, as the main electrode material or as an addition to increase electron transport between a target analyte and the electrode surface. Screen-printed graphene electrodes (SPGEs), in particular, have been shown to have a significantly greater electrochemical response than screen-printed carbon electrodes (SPCEs).
Graphene-based electrodes have also been modified using diverse materials, including metal nanoparticles, conductive polymers, and ionic liquids (ILs), to improve their electrochemical performance. The wide electrochemical potential window, strong solvation ability, low volatility, nontoxicity, and great chemical stability of ILs make them particularly intriguing.
Furthermore, because ILs are liquid-state salt compounds generated by coupling positive and negative ions, they have a high electrical conductivity. SPGEs and SPCEs surface-modified with imidazolium-type and pyridinium-type ILs, in particular, have demonstrated dramatically better electrochemical responses to a variety of analytes. Developing excellent electrochemical electrodes based on ILs and graphene is so promising.
As a result, screen-printed electrodes (SPEs) have seen much use in electrochemical studies. However, no electrodes directly manufactured by screen printing inks comprising ILs and GP have been reported. In terms of electrode homogeneity, reusability, and controllability, electrodes made by screen printing from mixes of ILs and GP should outperform IL-modified SPEs.
Disposable screen-printed IL/graphene electrodes (SPIL-GEs) were created in a new study published in the journal Electrochemistry Communications utilizing pastes made by mixing ILs with electrolytically exfoliated graphene and carbon paste (CP) in a ball mill.
Furthermore, one pyridinium-type and five imidazolium-type ILs were tested, and the IL concentration was modified to enhance the electrochemical sensing performance for the detection of three typical electroactive analytes: Ferri/ferro cyanide (Fe(CN)6)3-/4- redox pair, dopamine (DA), and hydroquinone (HQ). The shapes and sizes of the developed electrodes were also modified to provide the optimum electrochemical response.
Methodology
All compounds employed in this study were analytical grade and were not purified further. The electrolyte for electrolytic exfoliation was a commercial poly(3,4-ethylenedioxythiophene) polystyrene sulfonate (PEDOT/PSS) solution (Clevios P Jet N from HC Starck, USA).
To integrate and homogenize screen-printing inks, a commercial ball mill (Retsch model Emax) was employed. The conductive paste was printed on PET substrates using a screen printer (DEK model 03ix). Clicking voltammetry (CV) measurements were performed using a potentiostat (µ-Autolab Type III, Methrom) with the GPES software to investigate electrochemical performance.
As shown in Figure 1A, five geometric designs of electrodes were created using Adobe Illustrator, with varied total electrode lengths and active regions for the working electrode (WE) and counter electrode (CE).
As shown in Figure 1C, an electrical connection was used to connect the printed electrodes to the potentiostat and electrochemically scrubbed to remove impurities on electrode surfaces in 0.1 M PBS solution (pH 7.4) by a voltage applied between - 0.3 and + 0.6 V in cyclic voltammetry (CV) mode for five cycles at a scan rate of 50 mVs-1.
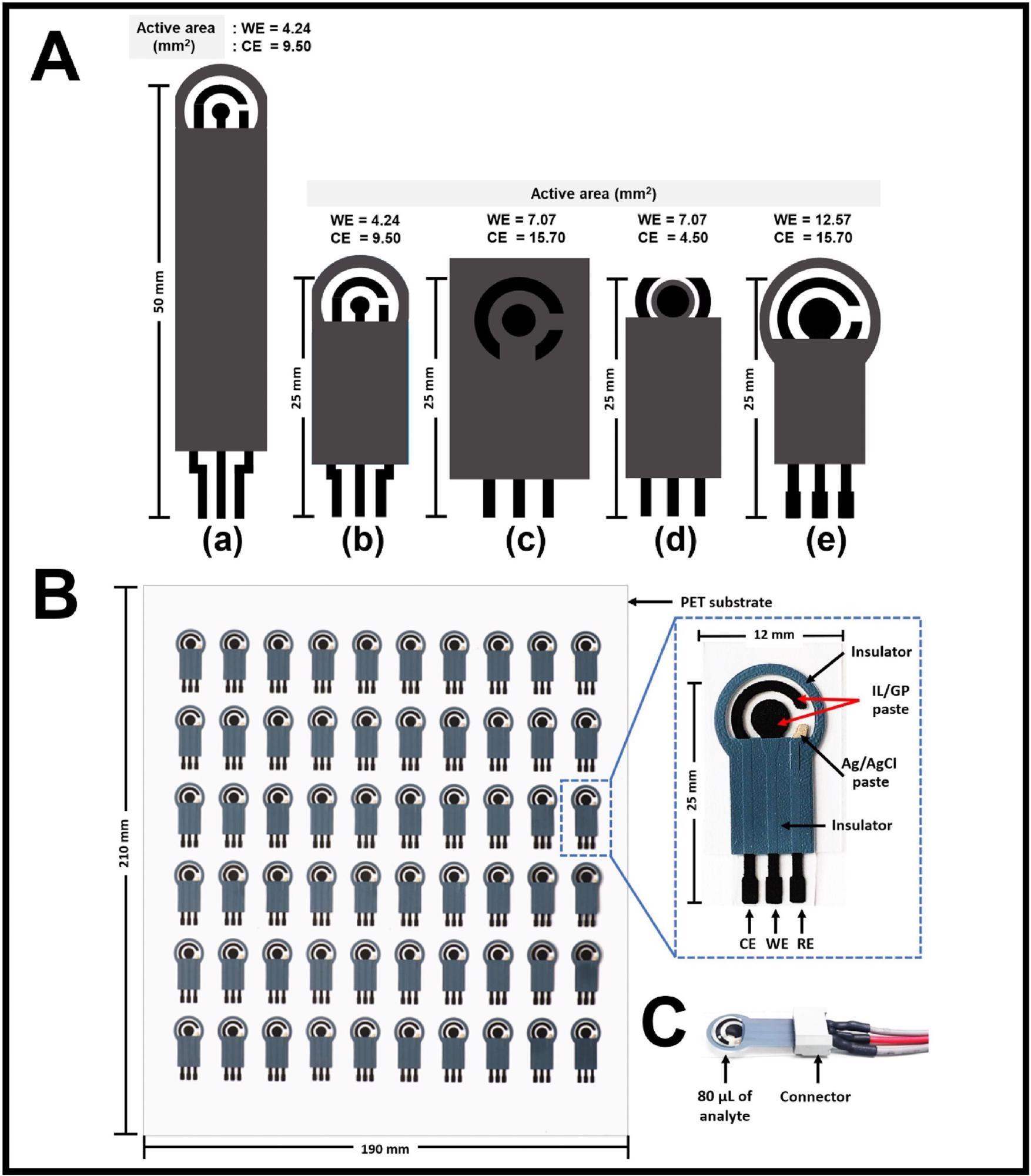
Figure 1. (A) Five designs of screen printed electrodes and typical photographs of (B) fabricated SPIL-GEs on a single PET substrate (190 × 210 mm2) and (C) a single SPIL-GE with an electrical connector. Image Credit: Kamsong, et al., 2022
Results
Figures 2A, 2B, and 2C show the impacts of three geometric factors on the CV response of SPGEs to 2.5 mM K3Fe(CN)6 in 0.1 M PBS solution (pH 7.4) at 50 mVs-1: total electrode length, WE, and CE active areas, respectively.
The electrochemical characteristics of all designed electrodes are also compared to theoretical ones by determining their electrochemical surface areas (ESAs) from CV currents utilizing the Randles–Sevcik equation (Ip = KACn3/2(Dv)1/2, where K = 2.69 105C V-1/2 mol-1, A = ESA (cm2), C = concentration (mol.cm-3), n = number of electron per reaction (=1 for Fe3+/4+), v = scan rate (Vs−1) and D = diffusion constant (=7.67 × 10-6 cm2s−1 for K4Fe(CN)6)) as combined with Jp are shown in Figure 2D.
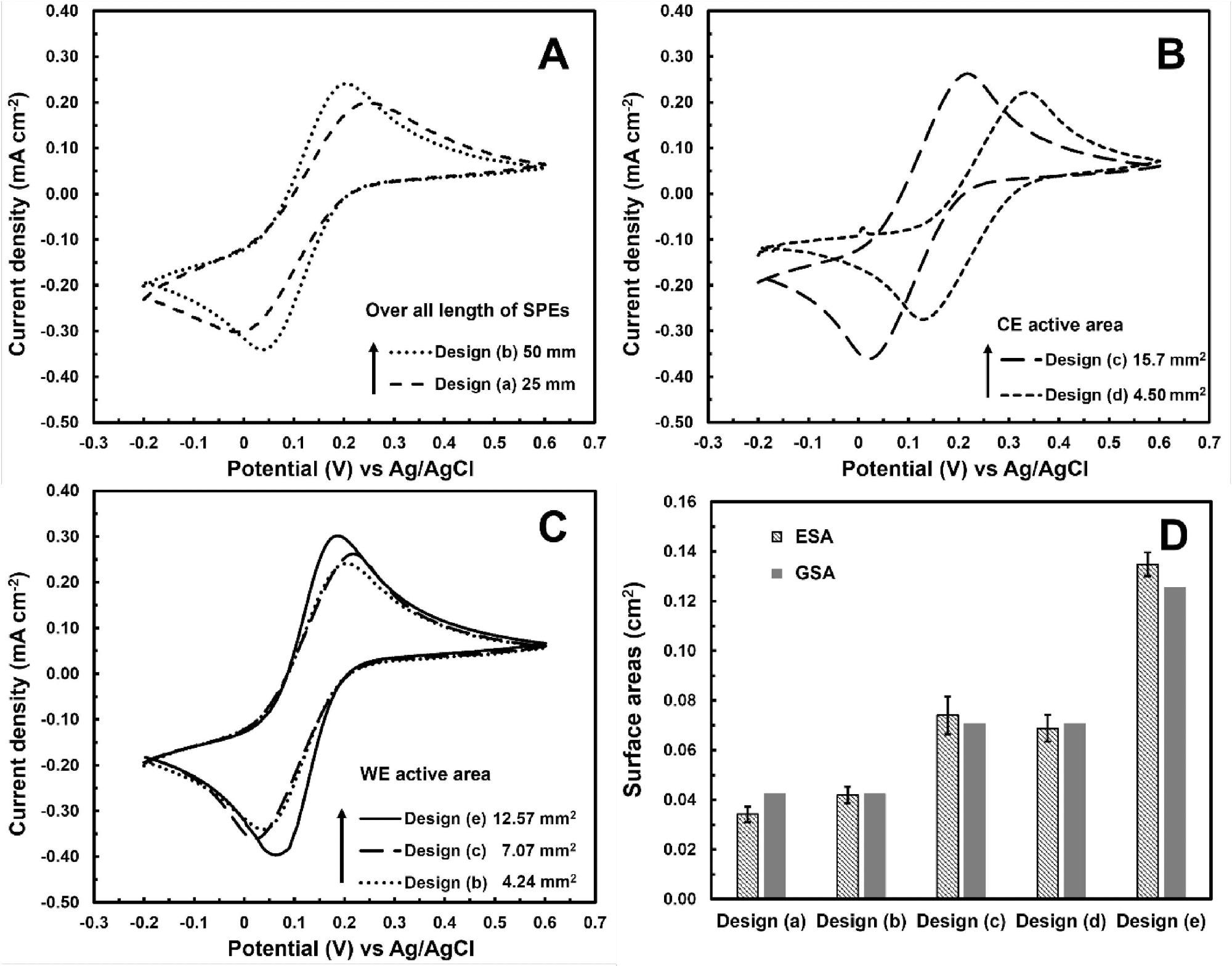
Figure 2. Cyclic voltammograms of SPGEs with different (A) overall electrode lengths (Designs (a)-(b)), (B) CE active area (Designs (c)-(d)) and (C) WE active area (Designs (b)-(c) and (e)) towards 2.5 mM K3Fe(CN)6 at 50 mVs−1. (D) Electrochemical and geometric surface areas (ESA & GSA) of SPGEs with different designs. Image Credit: Kamsong, et al., 2022
The electrochemical performance of SPIL-GEs with six IL materials, PMPlm, BMIMBF4, HMIMPF6, C9H15N3S, EMIMBF4, and BMIMPF6, was assessed by CV towards 5 mM K3Fe(CN)6 in 0.1 M PBS solution (pH 7.4) and compared to SPCE and SPGE, as shown in Figure 3A.
As shown in Figure 3B, the corresponding anodic peak amplitudes, as well as ESAs estimated using Randles–Sevcik, are displayed individually to examine the differences across all electrodes. The computed ESA values of SPIL-GEs are significantly greater than the GSA value, as seen in Figure 3C.
Figures 3C and 3D show the influence of PMPlm content varying from 0.5–2.0% (w/w) on CV response and anodic signals, as well as predicted ESAs of SPPMPlm-GEs towards 2.5 mM K3Fe(CN)6.
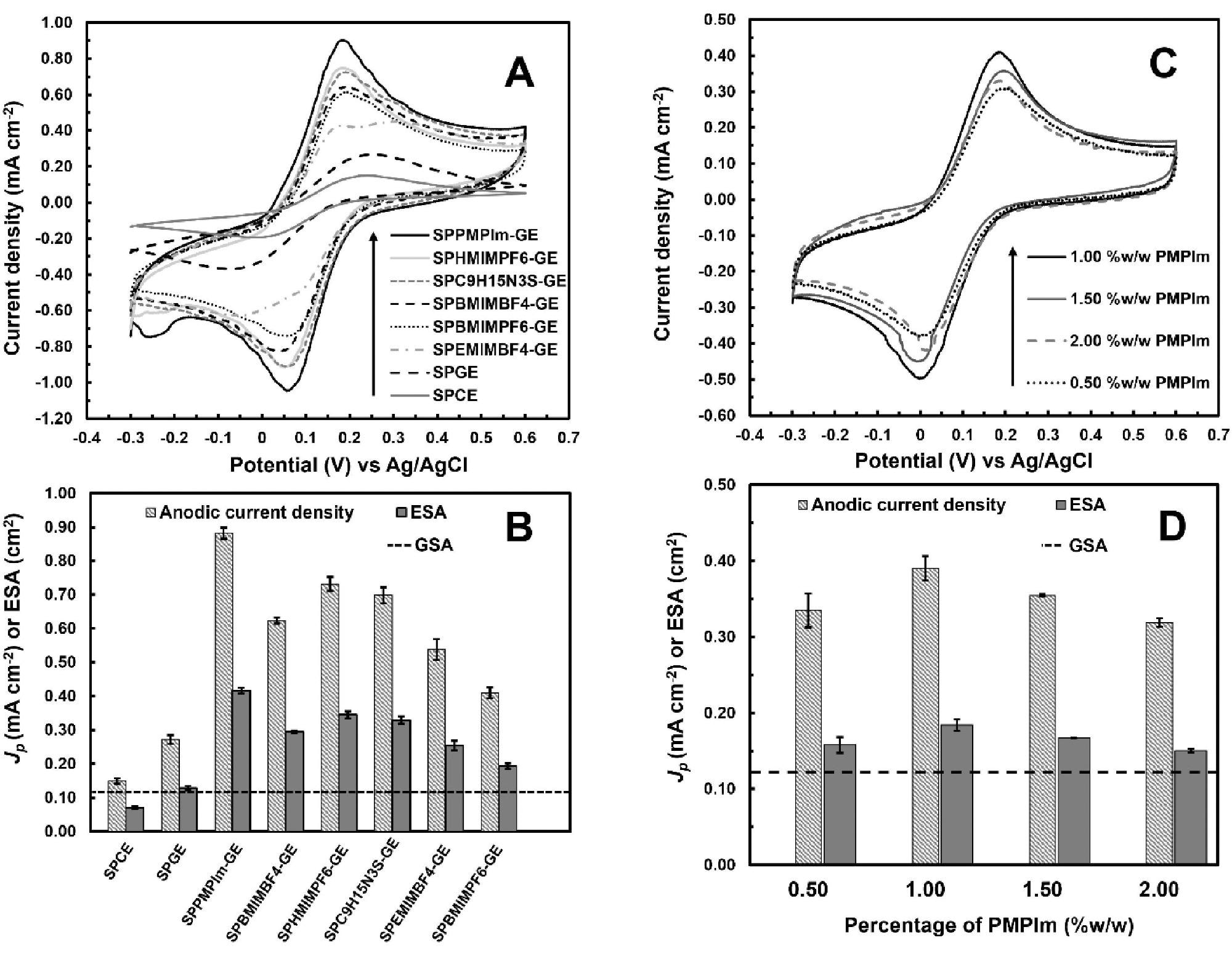
Figure 3. Cyclic voltammograms of (A) SPCE, SPGE, and SPIL-GEs with different IL materials towards 5 mM K3Fe(CN)6 at 50 mVs−1 and (B) corresponding Jp and ESAs. (C) Cyclic voltammograms of SPPMPlm-GEs with different PMPlm contents towards 2.5 mM K3Fe(CN)6 at 50 mVs−1 and (d) corresponding Jp and ESAs. The dotted lines in (B) and (D) correspond to the geometric surface area (GSA) of WE. Image Credit: Kamsong, et al., 2022
CV measurements in 2.5 mM K3Fe(CN)6, 1 mM DA, and 1 mM HQ solutions were used to analyze and compare the electrochemical performance of the optimum SPIL-GE with 1% PMPlm with that of SPGEs and SPCEs, as shown in Figure 4A-4C, respectively.
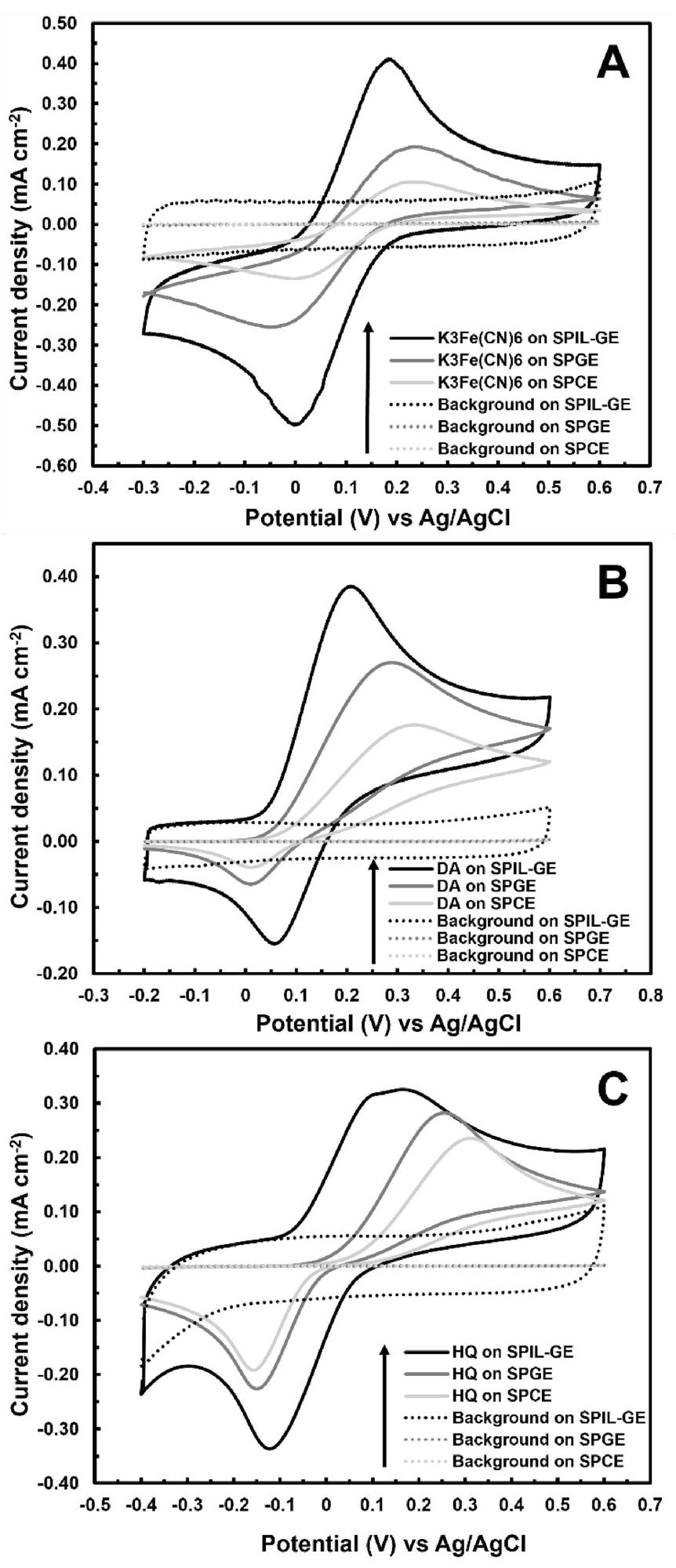
Figure 4. Cyclic voltammograms of SPIL-GE, SPGE, and SPCE towards (a) 2.5 mM K3Fe(CN)6, (b) 1 mM dopamine (DA) and (c) 1 mM hydroquinone (HQ) at 50 mVs−1. Image Credit: Kamsong, et al., 2022
Furthermore, as shown in Figure 5, the ideal SPIL-GE produces greater CV peak currents towards 2.5 mM K3Fe(CN)6 than two commercial electrodes (E1: graphene-modified SCPE and E2: SCPE).
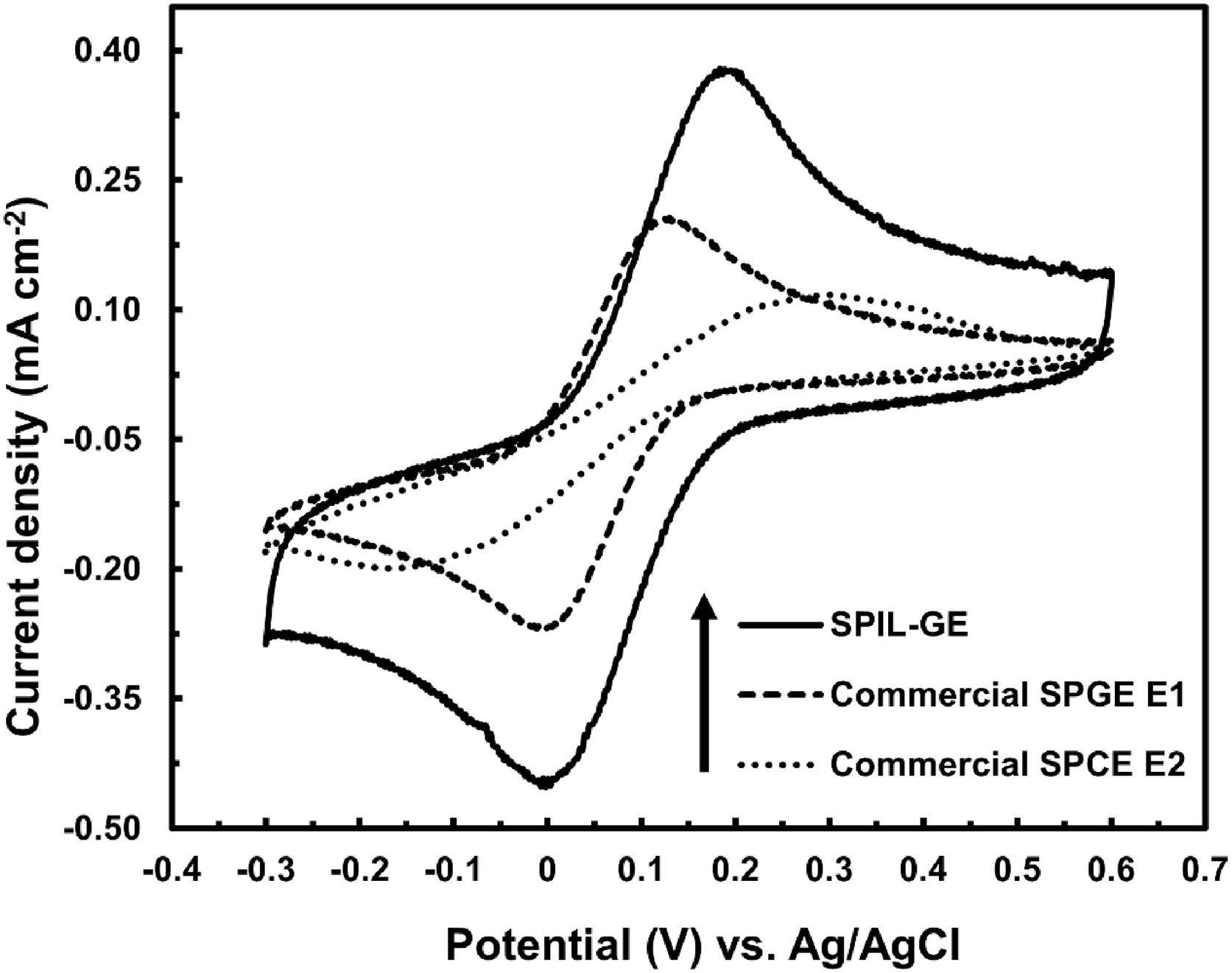
Figure 5. Cyclic voltammograms of SPIL-GE and two commercial SPGE E1 and SPCE E2 towards 2.5 mM K3Fe(CN)6 at 50 mVs−1. Image Credit: Kamsong, et al., 2022
FTIR spectroscopy was used to verify the chemical contents of SPIL-GE with the ideal IL (PMPlm) by comparing them to the spectra of its components, including PMPlm, GP/PEDOT/PSS, and CP, which is seen in Figure 6A. Figure 6B shows the surface morphologies of SPCE, SPGE, and SPIL-GE as determined by SEM.
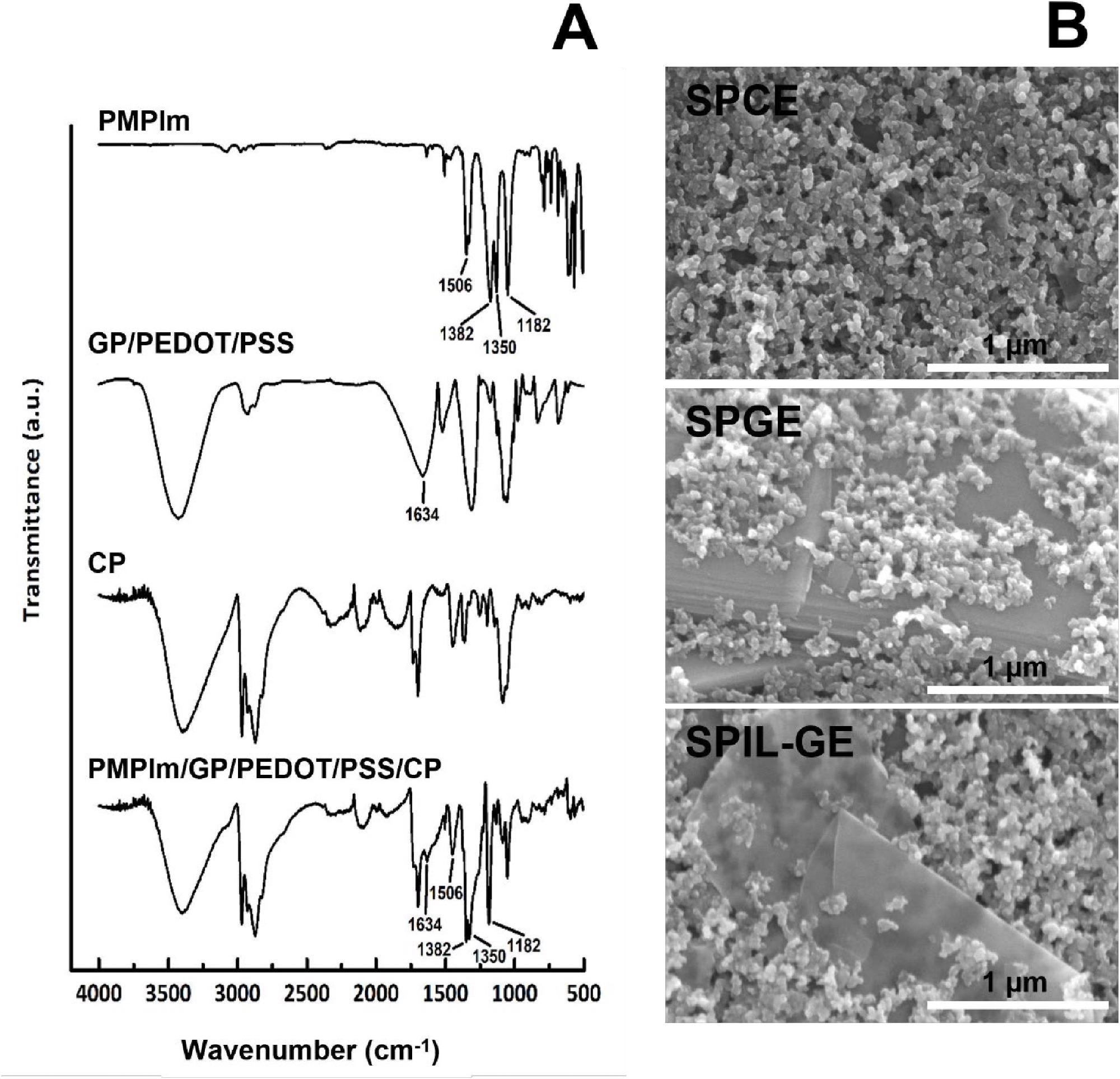
Figure 6. (A) FTIR spectra of PMPlm, GP/PEDOT/PSS, CP and PMPlm/GP/PEDOT/PSS/CP. (B) SEM micrographs of SPCE, SPGE and SPIL-GE. Image Credit: Kamsong, et al., 2022
Conclusion
SPIL-GEs were created by mixing graphene and ILs with commercial CP to create conductive inks. For SPGEs with different overall electrode lengths, as well as WE and CE active areas, CV measurements were used to improve electrode geometries towards (Fe(CN)6)3-/4-. According to the CV results, the best design had a total length of 25 mm and active areas of 12.57 and 15.7 mm2, respectively.
The electrochemical reaction of SPIL-GEs made of six IL materials, including PMPlm, HMIMPF6, BMIMBF4, C9H15N3S, EMIMBF4, and BMIMPF6, to K3Fe(CN)6 was investigated. At the ideal PMPlm level of 1.0% (w/w), PMPlm produced the greatest CV peak currents when compared to other ILs.
The electrochemical response of the optimum SPIL-GE was compared to SPCEs and SPGEs for three common electroactive analytes: (Fe(CN)6)3-/4- redox couple, DA, and HQ. For the three analytes, the results showed that SPIL-GE had higher oxidation currents and lower anodic potentials than SPGE and SPCE. As a result, the SPIL-GE may be a viable option for advanced electrochemical sensing applications.
Journal Reference:
Kamsong, W., Primpray, V., Pasakon, P., Sriprachuabwong, C., Pakapongpan, S., Mensing, J.P., Wisitsoraat, A., Tuantranont, A. and Karuwan, C., 2022. Highly sensitive and disposable screen-printed ionic liquid/graphene based electrochemical sensors. Electrochemistry Communications, p.107209. Available Online: https://www.sciencedirect.com/science/article/pii/S138824812200011X.
References and Further Reading
- Ambrosi, A., et al. (2016) Graphene and its electrochemistry – an update. Chemical Society Reviews, 45(9), pp. 2458–2493. doi.org/10.1039/C6CS00136J.
- Pumera, M (2010) Graphene-based nanomaterials and their electrochemistry. Chemical Society Reviews, 39(11), pp. 4146–4157. doi.org/10.1039/C002690P.
- Karuwan, C., et al. (2013) A disposable screen printed graphene–carbon paste electrode and its application in electrochemical sensing. RSC Advances, 3(48), pp. 25792–25799. doi.org/10.1039/C3RA44187C.
- Pasakon, P., et al. (2019) A high-performance, disposable screen-printed carbon electrode modified with multi-walled carbon nanotubes/graphene for ultratrace level electrochemical sensors. Journal of Applied Electrochemistry, 49, pp. 217–227. doi.org/10.1007/s10800-018-1268-1.
- Karuwan, C., et al. (2017) Screen-printed graphene-based electrochemical sensors for a microfluidic device. Analytical Methods, 9(24), pp. 3689–3695. doi.org/10.1039/C7AY00379J.
- Wisitsoraat, A., et al. (2013) Graphene–PEDOT:PSS on screen printed carbon electrode for enzymatic biosensing. Journal of Electroanalytical Chemistry, 704, pp. 208–213. doi.org/10.1016/j.jelechem.2013.07.012.
- Li, C & Shi, G (2011) Synthesis and electrochemical applications of the composites of conducting polymers and chemically converted graphene. Electrochimica Acta, 56(28), pp. 10737–10743. doi.org/10.1016/j.electacta.2010.12.081.
- Yang, W., et al. (2015) Flexible conducting polymer/reduced graphene oxide films: synthesis, characterization, and electrochemical performance. Nanoscale Research Letters, 10, p. 222. doi.org/10.1186/s11671-015-0932-1.
- Xue, Y., et al. (2011) The comparison of different gold nanoparticles/graphene nanosheets hybrid nanocomposites in electrochemical performance and the construction of a sensitive uric acid electrochemical sensor with novel hybrid nanocomposites. Biosensors and Bioelectronics, 29(1), pp. 102–108. doi.org/10.1016/j.bios.2011.08.001.
- Gupta, P., et al. (2018) An electrochemical aptasensor based on gold nanoparticles and graphene oxide doped poly(3,4-ethylenedioxythiophene) nanocomposite for detection of MUC1. Journal of Electroanalytical Chemistry, 813, pp. 102–108. doi.org/10.1016/j.jelechem.2018.02.014.
- Donini, C. A., et al. (2018) Reduced graphene oxide modified with silver nanoparticles for the electrochemical detection of estriol. Journal of Electroanalytical Chemistry, 809, pp. 67–73. doi.org/10.1016/j.jelechem.2017.12.054.
- Noor, A. a. M., et al. (2016) Microwave synthesis of reduced graphene oxide decorated with silver nanoparticles for electrochemical determination of 4-nitrophenol. Ceramics International, 42(16), pp. 18813–18820. doi.org/10.1016/j.ceramint.2016.09.026.
- Valentini, F., et al. (2015) Graphene and ionic liquids new gel paste electrodes for caffeic acid quantification. Sensors and Actuators B: Chemical, 212, pp. 248–255. doi.org/10.1016/j.snb.2015.02.033.
- Liu, R., et al. (2016) A graphene/ionic liquid modified selenium-doped carbon paste electrode for determination of copper and antimony. Analytical Methods, 8(5), pp. 1120–1126. doi.org/10.1039/C5AY02945G.
- Shabani-Nooshabadi, M., et al. (2020) Graphene oxide/NiO nanoparticle composite-ionic liquid modified carbon paste electrode for selective sensing of 4-chlorophenol in the presence of nitrite. Journal of Molecular Liquids, p. 14687. doi.org/10.1016/j.molliq.2020.114687.
- Mazloum-Ardakani, M., et al. (2014) Application of graphene to modified ionic liquid graphite composite and its enhanced electrochemical catalysis properties for levodopa oxidation. Sensors and Actuators B: Chemical, 204, pp. 282–288. doi.org/10.1016/j.snb.2014.07.069.
- Du, M., et al. (2011) Ionic liquid-functionalized graphene as modifier for electrochemical and electrocatalytic improvement: comparison of different carbon electrodes. Analytica Chimica Acta, 690(2), pp. 169–174. doi.org/10.1016/j.aca.2011.01.051.
- Valentini, F., et al. (2012) Oxidized graphene in ionic liquids for assembling chemically modified electrodes: a structural and electrochemical characterization study. Analytical Chemistry, 84(13), pp. 5823–5831. doi.org/10.1021/ac301285e.
- Kubisa, P (2005) Ionic liquids in the synthesis and modification of polymers. Journal of Polymer Science | Part A: Polymer Chemistry, 43(20), pp. 4675–4683. doi.org/10.1002/pola.20971.
- Singh, S K & Savoy, A W (2020) Ionic liquids synthesis and applications: An overview. Journal of Molecular Liquids, 297, p. 112038. doi.org/10.1016/j.molliq.2019.112038
- Greer, A. J., et al. (2020) Industrial Applications of Ionic Liquids. Molecules, 25(21), p. 5207. doi.org/10.3390/molecules25215207.
- Watanabe, M., et al. (2017) Application of Ionic Liquids to Energy Storage and Conversion Materials and Devices. Chemical Reviews, 117(10), pp. 7190–7239. doi.org/10.1021/acs.chemrev.6b00504.
- Tajik, S., et al. (2014) The first electrochemical sensor for determination of mangiferin based on an ionic liquid–graphene nanosheets paste electrode. Ionics, 20(8), pp. 1155–1161. doi.org/10.1007/s11581-013-1063-2.
- Nasir, M Z M & Pumera, M (2016) Impact electrochemistry on screen-printed electrodes for the detection of monodispersed silver nanoparticles of sizes 10–107 nm. Physical Chemistry Chemical Physics, 18(40), pp. 28183–28188. doi.org/10.1039/C6CP05463C.
- Geto, A., et al. (2019) Electrochemical determination of bentazone using simple screen-printed carbon electrodes. Environment International, 129, pp. 400–407. doi.org/10.1016/j.envint.2019.05.009.
- Mistry, K., et al. (2015) Electrochemical Characterization of some Commercial Screen-Printed Electrodes in Different Redox Substrates. Current Science, 109(8), p. 1427.
- Zhang, F., et al. (2014) Reference and counter electrode positions affect electrochemical characterization of bioanodes in different bioelectrochemical systems. Biotechnology and Bioengineering, 111(10), pp. 1931–1939. doi.org/10.1002/bit.25253.
- Valentini, F., et al. (2015) Graphene and ionic liquids new gel paste electrodes for caffeic acid quantification. Sensors and Actuators B: Chemical, 212, pp. 248–255. doi.org/10.1016/j.snb.2015.02.033.
- Zech, O., et al. (2010) The Conductivity of Imidazolium-Based Ionic Liquids from (248 to 468) K. B. Variation of the Anion. Journal of Chemical & Engineering Data, 55(5), pp. 1774–1778. doi.org/10.1021/je900793r.
- Stoppa, A., et al. (2010) The Conductivity of Imidazolium-Based Ionic Liquids from (−35 to 195) C. A. Variation of Cation’s Alkyl Chain. Journal of Chemical & Engineering Data, 55(5), pp. 1768–1773. doi.org/10.1021/je900789j.
- Valentini, F., et al. (2012) Oxidized Graphene in Ionic Liquids for Assembling Chemically Modified Electrodes: A Structural and Electrochemical Characterization Study. Analytical Chemistry, 84(13), pp. 5823–5831. doi.org/10.1021/ac301285e.
- Maleki, N., et al. (2007) Investigation of the Role of Ionic Liquids in Imparting Electrocatalytic Behavior to Carbon Paste Electrode. Electroanalysis, 19(21), pp. 2247–2250. doi.org/10.1002/elan.200703952.
- Mazloum-Ardakani, M., et al. (2014) Application of graphene to modified ionic liquid graphite composite and its enhanced electrochemical catalysis properties for levodopa oxidation. Sensors and Actuators B: Chemical, 204, pp. 282–288. doi.org/10.1016/j.snb.2014.07.069.
- Wang, Z., et al. (2014) Electrochemical determination of lead and cadmium in rice by a disposable bismuth/electrochemically reduced graphene/ionic liquid composite modified screen-printed electrode. Sensors and Actuators B: Chemical, 199, pp. 7–14. doi.org/10.1016/j.snb.2014.03.092.
- Wei, D i & Ivaska, A (2008) Applications of ionic liquids in electrochemical sensors. Analytica Chimica Acta, 607(2), 126–135. doi.org/10.1016/j.aca.2007.12.011.